Chapter 6
Light-Driven Chiral Molecular Switches or Motors in Liquid Crystal Media
6.1 Introduction
Thorough understanding and/or mimicking nature's art of expressing and augmenting chirality from microscopic to mesoscopic levels remains elusive. However, the ubiquitous bio-molecular self-assembly into helical structures such as the double helix of DNA, α-helix of peptides, and the elegant colors of butterfly wings, bird feathers, and beetle exoskeletons [1–4] has inspired chemists to develop novel materials not only to reveal the structure–property correlation but also to explore their usage in diverse technological applications. The foremost objective of such studies has been the design and synthesis of chiral molecular systems capable of yielding complex large scale helical structure originating from the manifestation of chirality in the constituent molecules through non-covalent supramolecular interactions. Among the self-organized supramolecular systems, liquid crystals (LCs) represent a promising class of materials which might exhibit stable supramolecular helical organizations if the mesogens are chiral. The fascinating helical superstructure of chiral nematic LCs, i.e., cholesteric LCs (CLCs), undoubtedly is a striking example of such self-organization owing to its unique property of selective reflection of light and its consequent potential applications. However, large scale production of chiral LCs with desired properties is discouraging because of the high cost of chiral starting materials, synthetic difficulties and purification challenges, etc. The search for alternative ways of obtaining chiral nematic phase has led to the observation that when small quantities of chiral materials, i.e., chiral dopants, are dissolved in an achiral nematic LC (NLC), this results in a chiral nematic phase. One of the hallmarks of such systems is the amplification of molecular chirality by the anisotropic medium. To further elaborate its scope and add another dynamic quality to the LC system, the incorporation of switchable chiral dopants capable of shape change under the influence of external stimuli has attracted tremendous attention in the recent years. Such dopants are known as chiral molecular switches or motors [5], where molecules should own bistable structures, normally two isomers, which can be driven easily to convert from one state to another by various external stimuli [6]. Thereby the handedness or/and pitch length of the induced helical organization by chiral molecular switches or motors can be tuned and controlled. Compared with molecular switches or motors driven by electric and magnetic field, heat, chemical or electrochemical reaction, those capable of being driven by light possess advantages of ease addressability, fast response time, and potential for remote control in a wide range of ambient environment. Hence, the subject of this chapter is confined to the use of light as the controlling stimulus to accomplish dynamic reflection wavelength changes including the inversion of helical handedness in induced cholesteric LCs. The LC materials can be applied not only in novel LC photo displays but also in various non-display photonic applications, such as optical switches, optical storage, optical computing, and energy-saving devices. Effective materials for molecular switches or motors with chiral component(s) are being sought comprehensively as viable dopants for LCs in order to achieve complete light-driven systems for the applications above.
In this chapter, we will focus on light-driven chiral molecular switches or motors in LC media for the induction and manipulation of photoresponsive cholesteric LC system and their consequent applications.
6.2 Photoresponsive Cholesteric Liquid Crystals
Historically, chiral nematic LCs were called cholesterics because the first materials observed exhibiting this phase were cholesterol derivatives. Nowadays this is not the case and there exist many different types of chiral materials that exhibit chiral nematic (cholesteric) phase and most of them have no resemblance to cholesterol whatsoever. Cholesteric LCs have similar orientational order as nematics but differ from the fact that the molecules are locally oriented in a plane which rotates around a perpendicular direction (called helical axis) that repeats itself within a length called pitch. The pitch characterizes the distance across the helical axis where the director in each “plane” completes a full 360° rotation. For this reason, cholesterics may be picturized as a layered structure where the layer separation corresponds to half pitch, which is easily observed in the “finger print” texture of cholesterics.
As will be discussed later, the light reflections as well as any other applications are directly related to the pitch. Ever since the first application of cholesterics was discovered, being able to tune the pitch has been a major goal, as it would allow dynamic change in the system, for example, continuously changing the wavelength of reflected light. However, a direct tuning has always been an issue. Perhaps the easiest and most widely used manner is by taking advantage of photoresponsive CLC materials where light-driven molecules suffer structure change under irradiation leading to change in the helical superstructure and therefore a shift in the pitch length. There are three basic methods to obtain photoresponsive cholesteric LCs. The first way, also the most direct way, is to use photoresponsive chiral mesogens which can furnish the chiral nematic LC phase [7]. However, this method has a major problem that the pitch in such single molecular system is usually tuned over a relatively narrow range and cannot match its physical properties required for device applications, so it is considered as the oldest but not a very useful strategy. The second way is to photosensitize either nematic LC host/system or chiral doped LCs, i.e., dope both chiral molecules and achiral photoresponsive molecules in a nematic host, or dope both photoresponsive achiral/chiral molecules and non-photoresponsive chiral molecules in a nematic host. This method uses the photoresponsive cholesteric LC with more than one dopant in the nematic host, which makes the CLC system more complicated and may alter the desired physical properties of LC host. It is worth noting here that commercial LC blends are often composed of many components. The third and most commonly used method is to dope a small amount of photoresponsive chiral trigger molecules (light-driven chiral dopant) into an achiral nematic LC host, which can self-organize into a helical superstructure. The changes of concentration or shape of chiral dopant upon light irradiation can easily induce pitch change (Figure 6.1). When the chiral dopant and the LC host are mixed together, they will self-organize into a helical superstructure, i.e., CLC phase, and most of the LC properties will not change significantly if the amount of the trigger dopant is small. Currently the third strategy is being studied widely, and the most important aspect of this method is that it is the chiral dopant on which the sign and the magnitude of the CLC pitch strongly depend. Regardless of the method of how the cholesteric phase is obtained, when light propagates through the CLC medium, it selectively reflects light of specific wavelength according to Bragg's law. The average wavelength λ of the selective reflection is defined by λ = np, where p is the pitch length of the helical structure and n is the average refraction index of the LC material. Hence by varying the pitch length of the CLCs upon light irradiation, the wavelength of the reflected light can be tuned, providing opportunities as well as challenges in fundamental science that are opening the door for many applications such as tunable color filters, tunable LC lasers, optically addressed displays, and biomedical applications.
Figure 6.1 A schematic mechanism of the reflective wavelength of light-driven chiral molecular switch or motors in achiral nematic LC media reversibly and dynamically tuned by light. (See the color version of this figure in Color Plates section.)
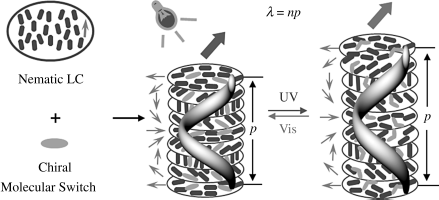
6.2.1 Helical Twisting Power of Chiral Dopants
As discussed above, while the cholesteric LC phase can be observed in single component molecular system, these materials are mostly formed by adding a chiral dopant to an achiral nematic LC host/system. When a chiral dopant is dispersed into a nematic LC media, the system self-organizes into a unique helical superstructure. The ability of a chiral dopant to twist an achiral nematic phase is expressed by the equation: β = (pc)−1 where β is helical twisting power (HTP), p is the pitch length of the helical structure, and c is the concentration of the chiral dopant in LC. Different dopant molecules have different capability to twist the NLC. Therefore, HTP is an important parameter for the applications of CLC systems.
So far, many different techniques have been developed to quantitatively measure the HTP of different dopant materials. However, there are two conventional techniques that are widely used nowadays. One is spectroscopic method, and the other is the Grandjean–Cano method [8–11]. The spectroscopic technique is mainly based on the unique reflection wavelength, which is governed by the equation: λ = np. Typical NLC host has an average refractive index that is around 1.6. Thus pitch length can be obtained by measuring the reflection wavelength of CLC. With known concentration c, β can be easily calculated here according to the equation: β = (pc)−1. The non-spectroscopic technique is usually based on a wedge cell, where the alignment is planar and substrates are rubbed parallel. The total twist inside the cell must be a multiple of half pitch in order to follow the boundary conditions. Thus the pitch is discrete and only certain pitch lengths are allowed. As the cell thickness change in the wedge cell, more half pitch turns are formed, but only when the cell gap and the boundary conditions allow it, as shown in Figure 6.2. This arrangement produces disclination lines between areas that contain a different number of layers. The disclination lines of CLCs in the wedge cell can be observed through a polarizing optical microscope. The pitch can be determined according to the equation: p =2R tan θ, where R represents the distance between the Grandjean lines and θ is the wedge angle of wedge cells. The inverse of pitch proportionately increases with increasing the concentration of the chiral dopant and HTP value.
Figure 6.2 Schematic illustration of a Grandjean–Cano wedge cell for the HTP measurement of cholesteric LC. Disclination lines are pointed out with arrows and the thickness change between two domains is marked p/2.
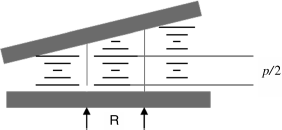
6.3 Light-Driven Molecular Switches or Motors as Dopants
Chiral dopants for LC research have been developed mainly for two different purposes. The first purpose focuses on the development of chiral dopants with persistent shape, and the research mainly aims at achieving high HTP and investigation of the interaction between chiral dopant and LC host molecules [12–14]. Another purpose, currently attracting more attention, is to develop switchable chiral dopants, whose shapes are changed by external stimulus such as light or heat [15–18]. Such molecular switches can act much as an electronic “on and off” switch under light-driven condition. These molecules can exist in at least two stable states and the equilibrium of the transition between these two states can be achieved upon light irradiation as shown in Figure 6.3. Moreover, light-driven switching requires that the photoresponsive molecule employed as chiral dopant either reverses its intrinsic chirality or forms different switching states capable of inducing the helical superstructure including handedness inversion of cholesteric helix upon light irradiation.
Figure 6.3 Schematic representation of a light-driven bistable switch.
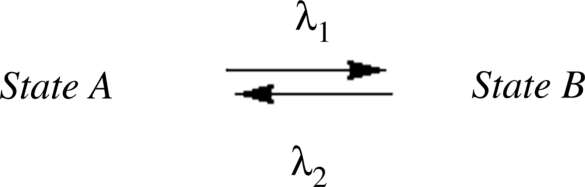
Many different molecular switchable systems based on azobenzene, spiropyran, fulgide, diarylethene, etc. have been developed [19, 20]. These chiral molecular switches can be applied as bistable dopants for switching in LC media to create different helicity and pitch length in cholesteric states. As mentioned above, a variety of external stimuli, including pH, pressure, magnetic field, solvent, chemical reactions, electric field, heat, and light can induce the switching process [21]; however, heat and light are most commonly applied for these LC systems due to their non-destructive, reversible nature. Light especially has advantages over other stimuli, and can be used at selected wavelengths, distinct polarizations, and different intensities as well as for remote, spatial, and temporal controls. Moreover, the use of photoresponsive chiral dopants in optically addressed displays would require no drive electronics or control circuitry and can be made flexible. Furthermore, it gives the possibility of laser and mask applications, as the radiation pattern and intensity distribution can be accurately controlled. As a result, most of the molecular switches are designed as light-driven switches, which are doped into an LC media to achieve the change in helical pitch or order upon irradiation with the appropriate wavelength of light. Light-driven chiral switches or motors doped in LC media can be classified and distinguished by the different radiation triggered processes.
The first report on modulation of CLC properties by doping photoresponsive materials was reported by Sackmann in 1971 [22], where azobenzene was chosen as the photo trigger molecule. After that initial study, many molecular switches or motors were applied as light-controlled dopants in LC media. All these switches or motors exist as bistable structures; however, molecules with bistable states cannot necessarily be used as chiral dopants. As a result, the molecules that are regarded as light-driven chiral molecular switches or motors in LCs should possess the following properties. First and foremost, the chiral switch or motor must be soluble in LC host. It must maintain light stability as well as light sensitivity in the host material. The switch or motor must have an adequately high HTP to induce a Bragg reflection since its high concentration can often lead to phase separation, coloration, and alter the desired physical properties of the LC host. The excitation and relaxation in the host material must be tunable with fatigue resistance. Accordingly, many molecular switches or motors have been developed especially over the past decade and are widely used as light-driven chiral dopants in LC media to induce the photoresponsive CLCs, which are illustrated and discussed in the following sections.
6.3.1 Chiral Azobenzenes as Dopants
Azobenzenes have the unique feature of reversible trans–cis isomerization upon light irradiation, which can cause the large conformational and polarization changes intramolecularly. The trans-form of azobenzene has a rod-like structure that can stabilize the LC superstructure, whereas its cis-form is bent-like structure and generally destabilizes the LC superstructure by generating disorder in the aligned systems. This property has been used in photochemical orientation of nematic films [23–25], pitch change in cholesterics [23–29], and phase transitions from nematic to isotropic states [30]. The dopant containing an azobenzene core which effects a change in cholesteric pitch upon irradiation was first reported in 1971 [22]. However, the azobenzene moiety is still the most widely used photoactive bistable group in LC research today because of its easy synthesis and having a good compatibility with LC phase especially in its trans-form (its elongated structure). Besides, due to the dramatic difference of molecular geometry of trans- and cis-forms, the HTPs of these states typically have large difference, which in turn makes a large change of the cholesteric pitch.
Generally in a CLC mixture containing chiral azobenzene, the HTP of chiral azobenzene dopant depends on its molecular structure, the nature of chirality, and the interaction with host molecules [31]. It is interesting that azobenzene with axial chirality usually shows much more efficient ability to induce the cholesteric LC phase than azobenzene with tetrahedral chirality. For example, the highest HTP (β) values reported for azobenzenes with tetrahedral chirality are around 15 μm−1 [31–36], whereas azobenzenes with axial chirality can have β value over 300 μm−1 [36–39] (Figure 6.4).
Figure 6.4 Molecular structures of chiral azobenzenes 1 and 2, and their associated HTPs.
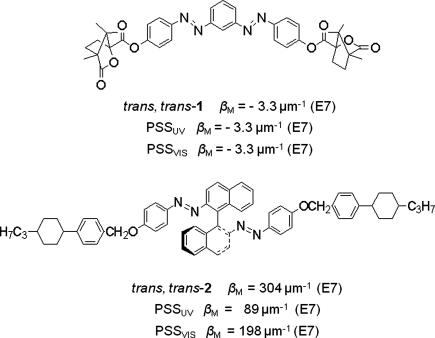
It is known that the trans-isomer of chiral azobenzene normally shows more efficient cholesteric induction than its cis-form, whereas even small amounts of its cis-forms can destabilize the LC phase into an isotropic phase. For example, Li et al. reported chiral azobenzene 3 with tetrahedral chirality as a mesogenic dopant in nematic LC 5CB (Figure 6.5) [40]. As expected, its HTP is low, which is approximately 13 μm−1. The chiral mesogenic dopant 3 needs to dope 25 wt% into an achiral nematic 5CB (or K15) to induce phase chirality with characteristic fingerprint texture (Figure 6.5A). Within 10 s under UV irradiation, this sample transits to isotropic phase as evidenced by a texture change as shown in Figure 6.5B. This experiment exhibited that the conversion from trans to cis configuration of the chiral dopant resulted in destabilization of the LC phase of the mixture. Removal of UV light immediately led to reverse process of chiral nematic domain formation from isotropic phase appearing as droplet nucleation followed by coalescence (Figure 6.5C). The reversion to the original polygonal fingerprint texture in Figure 6.5A was reached within approximately 2 h at room temperature in the dark.
Figure 6.5 Crossed polarized optical micrograph of the mixture of 25 wt% 3 in an achiral nematic LC host 5CB on cooling at 38.9°C (A: before UV irradiation; B: after UV irradiation for 10 s; C: 20 s after removal of UV light at isotropic phase). Used with permission from Ref. [40].
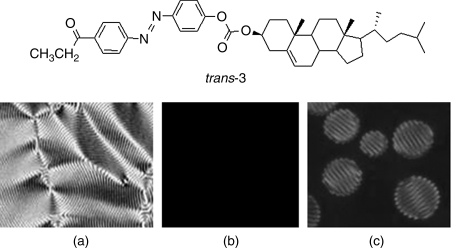
However, Ichimura et al. reported that the cis-forms of chiral azobenzenes 4–6 exhibited higher “intrinsic” HTPs than their corresponding trans-isomers (Figure 6.6) [41, 42], which might result from the cis-isomers having a more rod-like shape compared with their trans-forms.
Figure 6.6 Azobenzenes 4–6 with tetrahedral chirality.
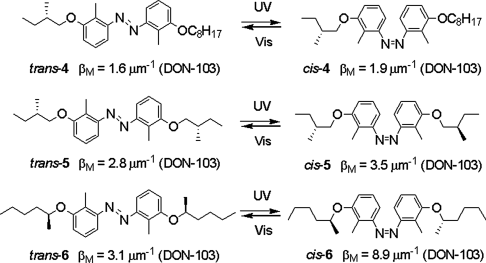
As mentioned above, HTP value of trans-chiral azobenzene is usually larger than that of its cis-chiral azobenzene. The combination of chiral azobenzene and non-photoresponsive chiral compound in nematic LC host can provide some interesting mechanisms for photochemical control of the helical structure such as phototuning helical pitch in any direction longer or shorter, phase transition between nematic and cholesteric phase, and handedness change of helical superstructure. Kurihara et al. reported photo-controlled switching of the photoresponsive CLCs consisting of chiral azobenzene (S)-7 and non-photoresponsive chiral dopant (S)-8 or its enantiomer (R)-8 (Figure 6.7) [35]. Chiral azobenzene (S)-7 induced a left-handed helix from an achiral nematic E44 whereas (S)-8 and (R)-8 induce a left- and right-handed helix, respectively. Figure 6.7a shows transmittance spectra of the CLC mixture of 17 wt% (S)-7 and 16 wt% (S)-8 in nematic LC E44 before and after UV irradiation, where the selective reflection wavelength was red-shifted upon UV irradiation. Contrary to the result shown in Figure 6.7a, the selective reflection of the CLC mixture composed of 5 wt% (S)-7 and 28 wt% (S)-8 in nematic LC E44 was blue-shifted upon UV irradiation. The results demonstrated that the helical pitch can be tuned and controlled in both directions to longer and shorter wavelengths by the combination of light-driven chiral switch or motor and non-photoresponsive chiral material as co-chiral dopant.
Figure 6.7 Top: Molecular structures of chiral azobenzene 7 and non-photoresponsive chiral dopant 8. Middle (a and b): Transmittance spectra of the mixtures consisting of photoresponsive chiral dopant 7 and non-photoresponsive chiral dopant 8 in nematic LC E44 before (solid lines) and after (dotted lines) UV irradiation [a: (S)-7/(S)-8/E44 17:16:67 in wt%; b: (S)-7/(R)-8/E44 5:28:67 in wt%]. Bottom (c): Polarized optical micrographs of 11.6 wt% (S)-7 and 8.4 wt% (R)-8 in E44 upon UV and visible light irradiation at 30°C. The LC mixture was in a 5 μm glass cell without any alignment treatment. Used with permission from Ref. [35].
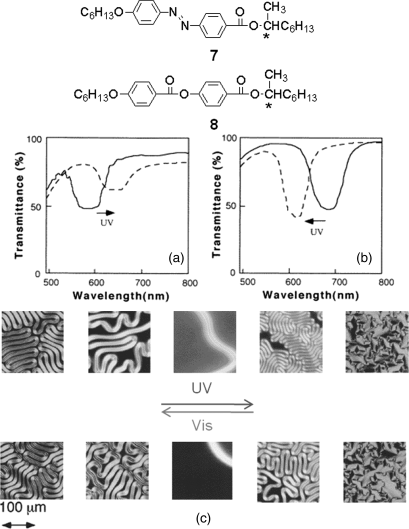
Kurihara et al. reported a combination of chiral azobenzene 9 and non-photoresponsive chiral compound 10 with LC host E44 to provide an effective photochemical modulation of the helical structure of CLCs (Figure 6.8) [43]. Non-photoresponsive chiral compound 10 was used for adjusting the initial reflection wavelength. Figure 6.9 (top) shows the colors reflected from the resulting CLC with different UV irradiation time. Before UV irradiation, the CLC was purple, and it turned to green, and then gradually to red with increasing irradiation time. The color could also be adjusted by varying the light intensity with a gray mask, as seen in Figure 6.9a and b. The resolution of the color patterning was estimated to be 70–100 μm by patterning experiments with the use of a photomask. The limitation of the resolution may be related to the diffusion of the low-molecular-weight compounds.
Figure 6.8 Molecular structures of chiral azobenzene 9 and non-photoresponsive compound 10.
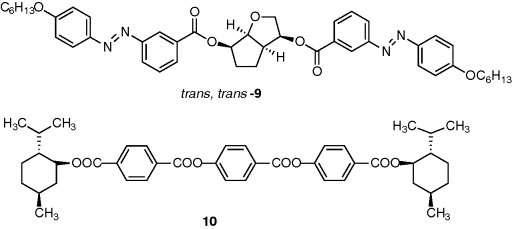
Figure 6.9 Changes in the reflection color of the CLC consisting of chiral azobenzene 9 and non-photoresponsive chiral dopant 10 in E44 by varying UV irradiation time: 0 s (left), 4 s (middle), and 10 s (right) (top); (a) gray mask and (b) red–green–blue (RGB) patterning of the CLC obtained by UV irradiation for 10 s through the gray mask at 25°C. Used with permission from Ref. [43]. (See the color version of this figure in Color Plates section.)
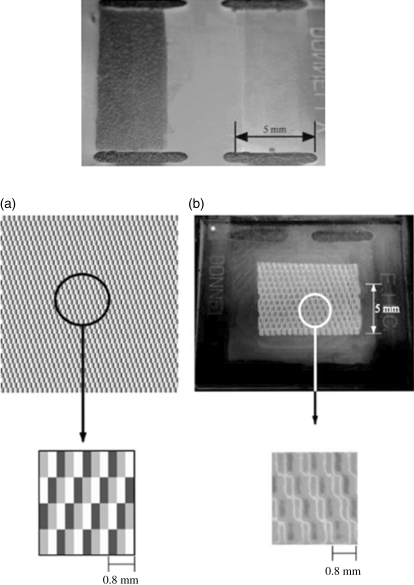
As mentioned previously, azobenzene with axial chirality usually induces short pitch cholesteric LCs due to high HTP. Many efforts were made to obtain a photo-controllable visible light reflector by doping axially chiral azobenzenes into a nematic LC media [44, 45]. The reflection wavelengths can be changed reversibly by photoisomerization of these azobenzenes [37, 38], normally red-shift upon UV irradiation and blue-shift upon visible light irradiation. Li et al. reported four reversible photoswitchable axially chiral azo dopants 11–14 with high HTPs as shown in Figure 6.10 [46]. These light-driven chiral switches were found suitable for dopants in nematic host for applications in novel optical addressed displays, i.e., photodisplay. For example, an image was created on the display cell filled with chiral switch 11-based CLC using UV light with a negative photo mask made of 10 mil PET placed on the top of the cell and exposed to UV light (637 μW/cm2 at λmax =365 nm) for 20 min. Depending on the optical density of the mask, certain areas were exposed with different intensities of light, resulting in an image composed of a variety of colors due to the various shifts in pitch length. Figure 6.10 (bottom) shows the photo of an original image (A), the negative mask (B), and the resulting image on the display cell (C). The light-driven switches in LC media were sufficiently responsive to an addressing light source that a high resolution image with gray scale could be imaged in a few seconds of irradiation time. It was further found that an image could be retained on the screen at room temperature for 24 h before being thermally erased. The high solubility of these materials in nematic host is also of commercial interest for stability in display applications.
Figure 6.10 Top: Molecular structures of light-driven switches 11–14 with axial chirality. Bottom: Illustration of an optically addressed image with negative photo mask [A: regular photograph of the original digital image; B: negative photo mask made of PET; C: image optically written on the display cell]. Used with permission from Ref. [46].
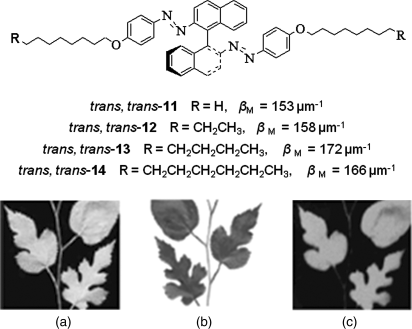
A flexible optically addressed photochiral display is shown in Figure 6.11A [47, 48]. This photochiral display is also based on reversibly photoswitchable axially chiral azobenzene 11 with high HTP and the ability for molecular conformational changes upon light irradiation [46]. This display is flexed and based on flexible cholesteric LC display technology [49, 50]. As shown in Figure 6.11, two identical displays were driven by different energies. One is electrically addressed with the standard multiplexing electronics, while the other one is optically addressed. Relatively, the overall size of the display module is reduced in case of the light-driven one and the cost can potentially be saved up to six times compared to the cost of the electric-driven one. The simplification of the final product can make markets such as security badges, small point of sale advertisements, and other applications that require a very low cost module that is updated infrequently now possible. It is worth noting here that the photo display device can display a high resolution image without the need of attached drive and control electronics, substantially reducing the cost of the display unit for use in applications where paper is currently used.
Figure 6.11 A flexible optically addressed photochiral display (A); a conventional display attached bulky and costly electronics compared with an optically addressed display with the same image without the added electronics (B). Used with permission from Ref. [47]. (See the color version of this figure in Color Plates section.)
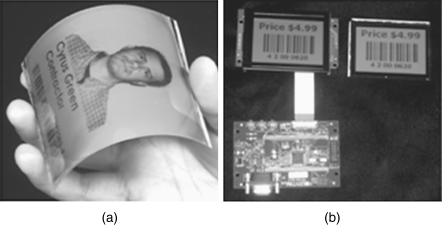
Phototuning reflection wavelength over 2000 nm was demonstrated by White et al. in an azobenzene-based CLC consisting of a high HTP axially chiral azobenzene 11 (Figure 6.12) [51]. Phototuning range and rate are compared as a function of chiral dopant concentration, light intensity, and thickness. CLCs composed of 11 maintain the CLC phase regardless of intensity or duration of exposure. The time necessary for the complete restoration of the original spectral properties (position, bandwidth, baseline transmission, and reflectivity) of 11-based CLC is dramatically reduced from days to a few minutes by polymer stabilization of the CLC helix.
Figure 6.12 Transmission spectra of 6 wt% 11 in LC1444 during phototuning for 5 μm thick cell. Used with permission from Ref. [51].
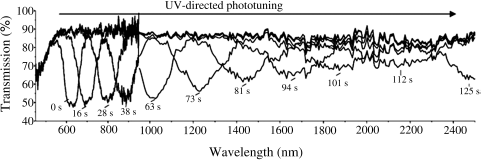
Green et al. reported two light-driven chiral molecular switches 15 and 16 with tetrahedral and axial chirality (Figure 6.13) [52]. When chiral switch 15 was doped in nematic LC host E31 at 15 wt% concentration, phototuning the reflection color over the entire visible region was observed. An amazing feature of this photoresponsive CLC system is quick relaxation. After 1 min of exposure to bright white light, it has surprisingly returned to the original ambient color. Unfortunately, the mixture in such high concentration is near saturation level and visible signs of phase separation after several phototuning cycles were observed due to their poor solubility in LC host.
Figure 6.13 Molecular structures of light-driven molecular switches 15 and 16 with tetrahedral and axial chirality. Used with permission from Ref. [52].
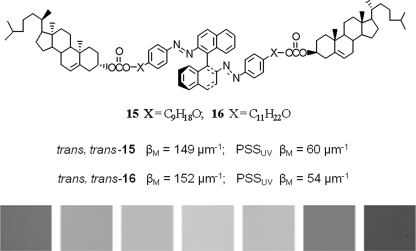
As noted before, light-driven switch 2 with axial chirality exhibited the highest HTP value for any light-driven switch reported so far [39]. The switch was found to be able to impart its chirality to a commercial nematic LC host, at low doping levels, to form a self-organized, optically tunable helical superstructure capable of fast and reversible phototuning of the structural reflection across entire visible region. This was the first report on reversible phototuning reflection color truly across entire visible region by employing light-driven chiral molecular switch or motor as the only chiral dopant in a LC media. For example, a mixture of 6.5 wt% 2 in nematic LC E7 was capillary filled into a 5 μm thick glass cell with a polyimide planar alignment layer and the cell was painted black on one side. The reflection wavelength of the cell was able to be tuned starting from UV region across the entire visible region to near infrared region upon UV irradiation at 365 nm (5.0 mW/cm2) within approximately 50 s, whereas its reversible process starting from near infrared region across the entire visible region to UV region was achieved by visible light at 520 nm (1.5 mW/cm2) or dark thermal relaxation. The reflection colors across the entire visible region were uniform and brilliant as shown in Figure 6.14A and B. Its ability to reversibly phototune the reflection color truly across entire visible region is further evidenced in Figure 6.14C and D. The reversible process with visible light is much faster than dark thermal relaxation. For instance, the phototuning time of 6.5 wt% 2 in E7 with a visible light at 520 nm (1.5 mW/cm2) from near IR region back across entire visible region to UV region is within 20 s whereas its dark thermal relaxation back through the entire visible region took approximately 10 h. Each reflection spectrum in Figure 6.14C and D has no drawback such as the dramatic change of the peak intensity and bandwidth compared with electric field-induced color tuning [53]. The reversible phototuning process was repeated many times without degradation. It is worth noting here that the reversible phototuning process across the entire visible region was achieved in seconds with the increase of light exposure intensity.
Figure 6.14 Reflection color images of 6.5 wt% chiral switch 2 in commercially available achiral LC host E7 in 5 μm thick planar cell. A: upon UV light at 365 nm (5.0 mW/cm2) with different time; B: reversible back across the entire visible spectrum upon visible light at 520 nm (1.5 mW/cm2) with different time. The colors were taken from a polarized reflective mode microscope. Reflective spectra of 6.5 wt% chiral switch 2 in LC E7 in a 5 μm thick planar cell at room temperature. C: under UV light at 365 nm wavelength (5.0 mW/cm2) with different time (3 s, 8 s, 16 s, 25 s, 40 s, and 47 s, from left to right). D: under visible light at 520 nm wavelength (1.5 mW/cm2) with different time (2 s, 5 s, 9 s, 12 s, and 20 s, from right to left). Used with permission from Ref. [39]. (See the color version of this figure in Color Plates section.)
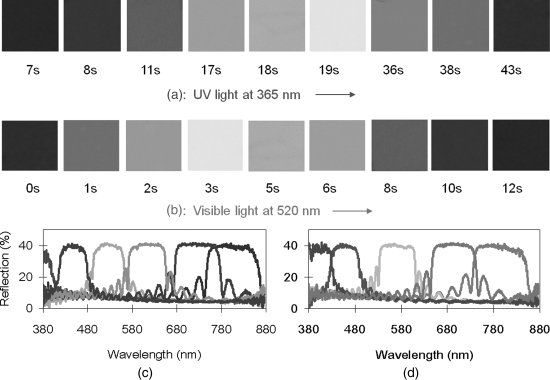
Furthermore, this chiral switch 2 was used in a color, photo-addressed liquid crystal display driven by light and hidden as well as fixed by application of an electric field from thermal degradation. Like conventional cholesteric LCs, the chiral switch doped in LC media is able to be electrically switched to bistable display by using polymer stabilized or surface stabilized chiral nematic texture. Even though the optically switched azo compounds are not thermally stable, an image can be made thermally stable and be retained indefinitely by electrically switching either the image or the image background to the focal conic state before it thermally relaxes. The image or its background is electrically selected by shifts in the electro-optic response curve that result from a change in the twisting power of the photosensitive chiral compound. An advantage of this display is that a thermally stable high resolution image can be captured without patterned electrodes or costly electronic drive and control circuitry, and retained indefinitely until electrically erased. Here such a light-driven device was made using the chiral switch 2. The phototunable cholesteric layer sandwiched between two simple unpatterned transparent electrodes is sufficient. For example, an optical writing took place within seconds in a planar state through a photomask by a UV light. The reflective image (Figure 6.15A) can be hidden in focal conic texture by applying a 30 V pulse and revealed by applying a 60 V pulse (Figure 6.15C). Moreover, by applying a 30 V pulse to an optically written image so as to make the UV irradiated region going to the focal conic texture and the UV un-irradiated region going to the planar texture, an optically written image can be stored indefinitely because the planar and focal conic textures are stable even though the light-driven switch relaxes to the un-irradiated state.
Figure 6.15 Images of 5 μm thick homeotropic alignment cell with 4 wt% chiral switch 2 in LC host E7. The image was recorded in a planar state through a photomask by a UV light (A). The image was hidden by a low voltage pulse in a focal conic state (B), which was reappeared by a high voltage pulse (C). The background color in the cell can be adjusted by light. Used with permission from Ref. [39]. (See the color version of this figure in Color Plates section.)
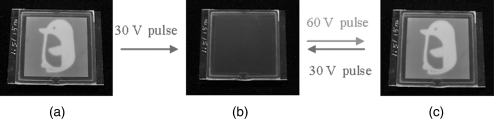
Chiral cyclic azobenzene switches have also been used to investigate the light-driven twisting behaviors for CLC system [54–56]. It was reported that some chiral cyclic compounds showed a reversible inversion in the handedness of CLC by means of their photoisomerization upon light. Manoj et al. recently reported a fast photon mode reversible handedness inversion of a self-organized helical superstructure, i.e., cholesteric LC phase, using light-driven chiral cyclic dopants (R)-17 and (R)-18 [56]. The two light-driven cyclic azobenzenophanes with axial chirality show photochemically reversible trans to cis isomerization in solution without undergoing thermal or photoinduced racemization (Figure 6.16A). The switches exhibited good solubility, high HTP, and a large change in HTP due to photoisomerization in three commercially available structurally different achiral LC hosts. Therefore, reversible tuning reflection colors from blue to near IR by light irradiation from the induced CLC was observed. More interestingly, the different switching states of the two chiral cyclic dopants were found to be able to induce a helical superstructure of opposite handedness. For example, a typical CLC texture observed for the N* phase of the CLC mixture containing 10 wt% (R)-17 in nematic LC ZLI-1132 under planar alignment conditions was quickly transformed into a planar N texture upon UV irradiation (Figure 6.16, A and B). As the sample in the N phase was rotated between fixed crossed polarizers, an extinguishing orientation of the cell was found when the orientation of the molecular director was along one of the polarizer directions (Figure 6.16C). This transient N phase was quickly transformed into an N* phase upon continued UV irradiation for a few more seconds (Figure 6.16D). The whole switching process was reversible with 440 nm irradiation. This provides clear evidence on the reversible handedness inversion upon light irradiation.
Figure 6.16 Top: Molecular structures of chiral cyclic azobenzenes (R)-17 and (R)-18 (A). Middle (B–D): Schematic mechanism of reflection wavelength tuning and handedness inversion of light-driven chiral molecular switch or motor in achiral nematic LC media reversibly and dynamically tuned by light. Bottom: Polarized optical photomicrographs of a planar aligned N* film containing 10 wt% (R)-17 in ZLI-1132 at room temperature, showing reversible phase transitions occurring by light irradiation of the sample inside a 5 μm cell: (a) oily streak texture of the N* phase before irradiation; (b) N phase obtained by exposure of the sample to UV irradiation; (c) extinguishing orientation of the N cell by rotation between crossed polarizers; (d) regeneration of the oily streak texture of the N* phase upon continued irradiation (bottom–right). Used with permission from Ref. [56]. (See the color version of this figure in Color Plates section.)
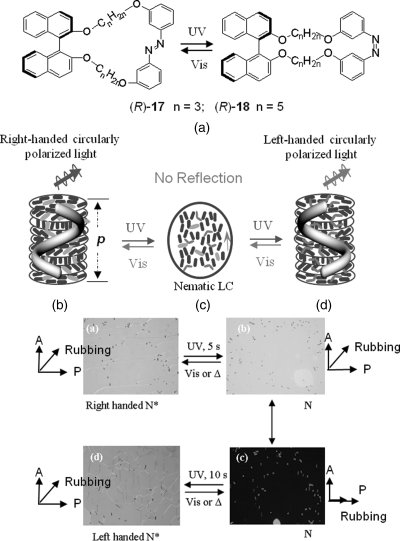
The induced helical pitch and photo-tunability of chiral cyclic dopants (R)-17 and (R)-18 in nematic LC media were measured using Cano's wedge method and the corresponding change in HTP values was summarized in Table 6.1. (R)-17 in its trans-form shows a high HTP value in E7 and K15 while the corresponding value in ZLI-1132 was found to be low. Its analog (R)-18 exhibits a lower HTP in E7 and K15 LC hosts compared to (R)-17. On the contrary, the HTP value of (R)-18 in ZLI-1132 was found to be higher than what was obtained for its lower homologue compound. Compared with its analog at ortho-substitution, the chiral switch (R)-17 with meta-substitution exhibited a higher HTP and a higher change in HTP, which might result from the intrinsic nature of its molecular structure and having a more dramatic geometrical change upon photoisomerization. Different LC hosts result in the different intermolecular associations between dopants and hosts. These results clearly reveal the subtle dependence of HTP on the molecular structures of both the dopant and the NLC hosts. Interestingly, Kawamoto et al. reported that (R)-17 can behave uniquely for non-destructive erasable chiroptical memory through its photoinduced switching in neat film [57].
Table 6.1 Helical twisting powers (β) of light-driven chiral molecular switches (R)-17 and (R)-18 in different nematic LC hosts as determined by Cano's wedge method and the observed change in values by irradiation. Positive and negative values represent right- and left-handed helical twists respectively.
6.3.2 Chiral Olefins as Dopants
Chiral olefins are the typical compounds with the capability of trans–cis isomerization similar to chiral azobenzenes, which can be used as light-driven chiral switches in LC media. Such compounds with exocyclic double bond should be chemically stable and do not form photo-dimers. However, to date only a few of these molecules have been reported to induce photoresponsive CLC system [58]. Yarmolenko et al. reported menthone-based chiral dopant 19 with high HTP and efficient cholesteric pitch modulation (Figure 6.17) [59]. Its cis-isomer was rather stable, and no thermally excited cis–trans isomerization was observed upon heating to 80°C, in contrast to azobenzene. As seen from Figure 6.17, the HTP value at its trans- and cis-form exhibited a considerable difference, which results from their dramatically different shape, similar to the change observed in azobenzene isomers. Chiral dopant 19 doped in nematic host MBBA exhibited a handedness inversion upon light irradiation, whereas no such handedness inversion of the resulting CLC was observed when using 5CB instead of MBBA as the nematic host. These results clearly reveal the subtle dependence of HTP on the molecular structure of nematic LC host since different LC host results in the different intermolecular association between dopant 19 and its host. The high HTP of 19 is probably due to its better compatibility and interaction in the LC medium owing to its very similar structure to the host LC molecules. Later, Lub et al. synthesized menthone derivatives 20 and 21 and observed moderate HTP in E7 mixture [60]. Moreover, in order to investigate the effect of chemical structure on HTP two new photoisomerizable compounds that are structurally related to menthone derivative 20 were designed and synthesized (Figure 6.17). However, the trans-isomers of nopinone and camphor derivatives 22 and 23 exhibited much lower HTPs than 20. It is possible that the chiral groups of the cage-like structure of 22 and 23 show less interaction with the LC host and hence lower HTP. It is interesting to note that the twist sense of the CLCs induced by 22 and 23 are opposite to the twist sense of 20. Furthermore, the twist sense of trans- and cis-isomers of 22 and 23 are also opposite. Though the HTPs are less for these compounds, their studies led to better understanding of structure–property relationship of chiral photoisomerizable dopants.
Figure 6.17 Menthone-based switchable chiral dopants.
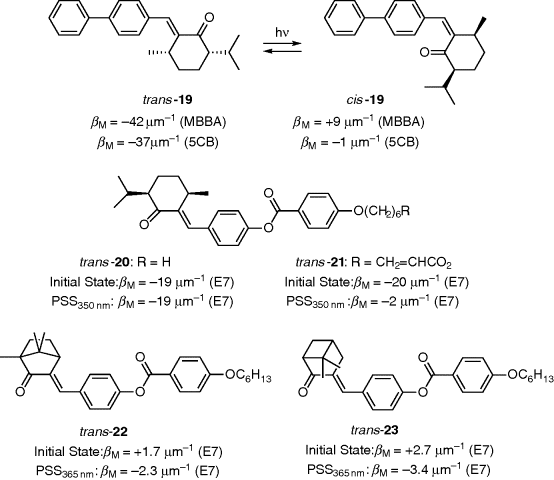
Stilbene derivatives are another class of olefins which undergo cis–trans isomerization upon photoirradiation. Therefore by linking chiral moieties, stilbenes can be made photoresponsive chiral dopants to induce chiral nematic phase and the pitch of the resulting phase can be modulated upon photoirradiation owing to their photoisomerization. Lub and co-workers have reported several chiral stilbene derivatives 24, 25 and 26 containing different chiral auxiliaries [61, 62]. Their structures and HTPs in achiral nematic liquid crystal hosts are shown in Figure 6.18.
Figure 6.18 Stilbene based switchable chiral dopants.
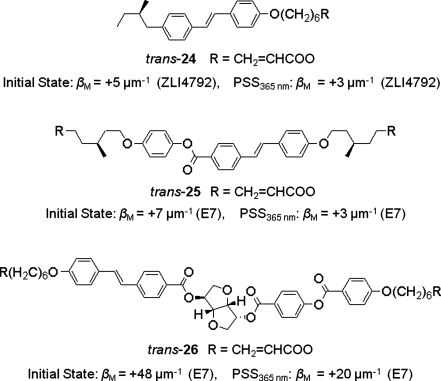
Similar to menthone and stilbene derivatives, cinnamic esters are also capable of exhibiting photoinduced cis–trans isomerization and hence are potential candidates for photoswitchable dopants. Accordingly several chiral cinnamate esters 27–30 (Figure 6.19) containing isosorbide as the chiral moiety have been synthesized and investigated as efficient chiral dopants in nematic LC media [63, 64].
Figure 6.19 Cinnamic esters based switchable chiral dopants.
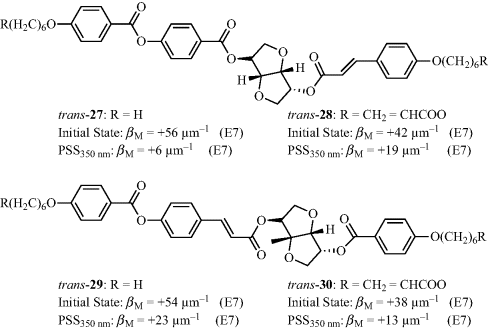
6.3.3 Chiral Diarylethenes as Dopants
Photochromic diarylethenes undergo a reversible 6-electron cyclization upon irradiation, leading to distinct change in structure and electronic configuration of the molecule [65]. This switching unit has been applied for reversible cholesteric to nematic transition and vice versa as well as photomanipulation of the cholesteric pitch [66–73]. Figure 6.20 shows some structures of these chiral diarylethenes. Feringa et al. reported the reversible cholesteric to nematic transition using open and closed form diarylethene 31 as shown in Figure 6.20 (top) [66]. When 1.4 wt% 31 in LC ZLI-389 was heated up under crossed polarizing microscope, a stable cholesteric phase was observed close to the N–I transition temperature. When the temperature was kept within the range of 51–54°C, the cholesteric phase with identical fingerprint texture was stable (Figure 6.20A). When it was irradiated with UV light at 300 nm for 50 s, the cholesteric phase disappeared and a nematic phase texture was observed (Figure 6.20B). Irradiation of the sample with visible light for 30 s resulted in the reappearance of the cholesteric fingerprint texture. This results from the fact that the open form of chiral diarylethene 31 facilitates the formation of a stable cholesteric phase in ZLI-389, while its HTP in the closed form is too low to effectively stabilize a cholesteric phase. Yamaguchi et al. reported photochromic diarylethene 32 with axial chirality which can induce a stable photoswitching between the nematic and cholesteric phase due to its very weak HTP (βM ~ 0 μm−1) at open form [68–72]. Cholesteric induction by this type of switch was supposed to be not very efficient because of extremely low HTP [74]. More recently, van Leeuwen et al. reported diarylethene 33 with a high HTP value of 50 μm−1 [73]. In contrast to the other diarylethene dopants reported previously, its ring-closed form 33 can induce CLC phase as well.
Figure 6.20 Top: Light-driven open-ring and closed-ring isomerization of photochromic chiral molecular switch 31; cholesteric fingerprint texture (A) and nematic texture (B) of 1.4 wt% 31 in ZLI-389 at 52°C. Molecular structure and HTP of photochromic chiral molecular switches 32 and 23. Used with permission from Ref. [66].
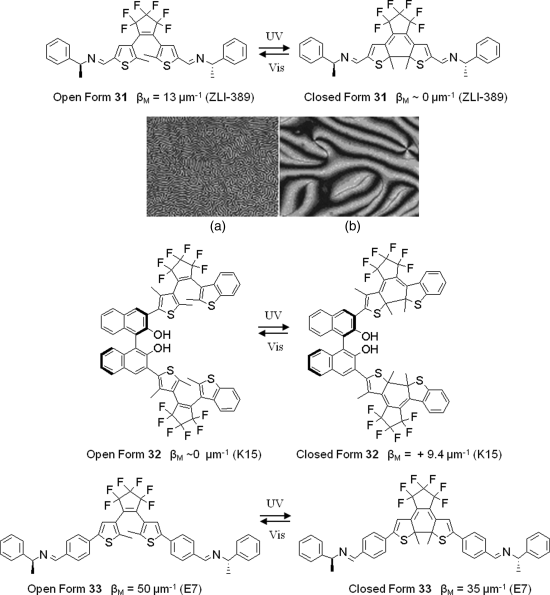
Rameshbabu et al. reported three photochromic chiral LC diarylethenes with tetrahedral chirality 34–36 which were found not only to be able to self-organize into a phototunable helical superstructure, but also to be able to induce a photoresponsive helical superstructure in an achiral LC host (Figure 6.21) [75]. For instance, 10 wt% 34 as a mesogenic dopant in a conventional achiral nematic 5CB exhibited a cholesteric polygonal fingerprint texture, as shown in Figure 6.21A. The transition from cholesteric to isotropic phase was observed. With UV irradiation at 310 nm (30 mW/cm2) for 30 s, it transformed into isotropic phase (Figure 6.21B) whereas upon visible irradiation at 670 nm the reverse process was observed, as evidenced by the formation of the chiral nematic domain from isotropic phase appearing as droplet nucleation followed by coalescence (Figure 6.21C). The reverse process upon visible light irradiation was reached within 30 min.
Figure 6.21 Molecular structures of chiral diarylethene 34–36 with tetrahedral chirality. Crossed polarized optical texture micrograph of 10 wt% of 34 in a nematic LC host 5CB before irradiation (A), after UV irradiation (B), and visible irradiation (C). Used with permission from Ref. [75].
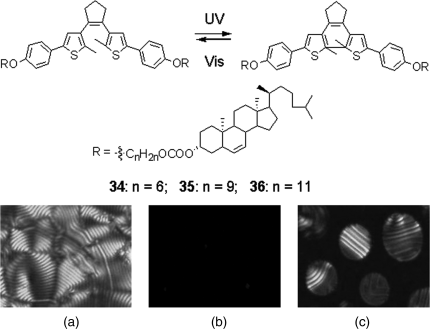
Very recently Li et al. reported three light-driven dithienylcyclopentene switches (S,S)-37, (R,R)-37, and (S,S)-38 (Figure 6.22) [76]. These chiral molecular switches with axial chirality were found not only to be able to act as a chiral dopant and induce a helical superstructure in an achiral nematic LC host, but also to be able to reversibly and dynamically tune the transmittance and reflection of the resulting cholesteric phase upon light irradiation. Light-driven chiral switch 37 exhibited an unusually high HTP which is significantly larger than those of the known chiral diarylethenes reported so far.
Figure 6.22 Diarylethenes 37 and 38 with axial chirality and their HTP values.
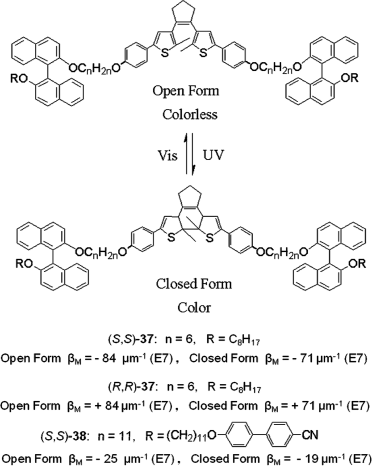
6.3.4 Chiral Spirooxazines as Dopants
Spirooxazine has been known as a promising photochromic compound with good photo-fatigue resistance for a long time [77]. Typical examples of photochromic reactions of spirooxazines are the reversible photochemical cleavage of the CߝO bond in the spirooxazine rings. Because the spiro-carbon of a spirooxazine molecule has potential as a chiral center, spirooxazines could be used as chiroptical molecular switches [78]. However, spirooxazines are usually racemic mixtures as shown in Figure 6.23. Therefore, if spirooxazines are to be used as chiroptical molecules in nematic LC system, modification of the spirooxazine with a chiral group is required. There are a few examples of spirooxazines used as the dopants in LC systems [78, 79]. Recently Jin et al. reported some novel thermally reversible photochromic axially chiral spirooxazines 40–43 [79]. These axially chiral spirooxazines showed ability to twist the nematic host LC E7 to form the cholesteric phases and the HTPs were relatively high (Figure 6.24). Additionally, the result illustrated that the chiral spirooxazines containing bridged binaphthyl moiety exhibit higher HTP than the corresponding unbridged ones either for the initial state (ring-closed form) or for the photostationary state (ring-opened form, irradiated with 365 nm UV light). Furthermore, this bifunctional system exhibited excellent thermally reversible photochromic behavior together with the chiral induction capability in LC hosts.
Figure 6.23 Schematic representation for the photochromic change of the spirooxazine 39.
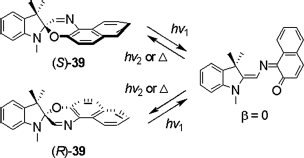
Figure 6.24 Molecular structures of light-driven spirooxazines with axial chirality 40–43 and their HTP values in E7.
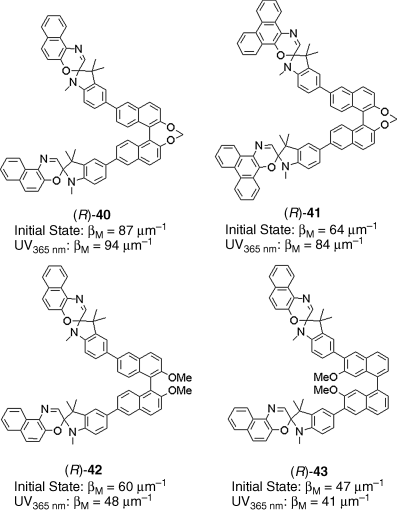
6.3.5 Chiral Fulgides as Dopants
Chiral fulgides are an interesting class of thermally irreversible photochromic materials with 6-electron cyclization upon light irradiation [80], which can be used as a light-driven trigger for LC systems. The photochromism of fulgides occurs between one of the colorless open forms and the photocyclized colored form. Yokoyama et al. reported that fulgides 44 and 45 with axial chirality acted as chiral dopants in nematic LC 5CB to induce cholesteric phase (Figure 6.25) [81–83]. The incorporation of an axially chiral binaphthol moiety into fulgide structure resulted in a bistable system with an enormous difference in HTP between the open and closed forms of the switch [81–83]. For example, chiral fulgide 45 at open form has a βM of −28.0 μm−1 in 5CB whereas its ring-closed isomer has an impressive βM of −175 μm−1. This allows photoswitching between cholesteric phases with a long and a short pitch, respectively, using small amounts of light-driven chiral dopant. The resulting CLC did not exhibit a handedness inversion upon light irradiation. However, this was circumvented with addition of non-photoresponsive chiral dopant (S)-dinaphtho [2,1-d:1′,2′-f] [1,3]dioxepin with opposite HTP (βM = +92 μm−1), resulting in reversible switching between a positive and negative handedness of cholesteric helix [83].
Figure 6.25 Molecular structures and photochromic reactions of indolylfulgides 44 and 45.
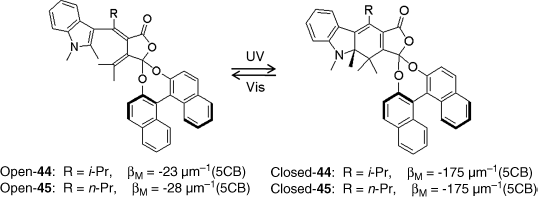
6.3.6 Chiral Overcrowded Alkenes as Dopants
Chiral overcrowded alkenes as dopants are much more likely to show inversion of the cholesteric helix sign upon switching. This kind of compounds is originally pioneered by Feringa and co-workers who continue to champion these materials for applications as molecular switches, molecular motors, and as enablers to photogenerate dynamic optical effects in CLCs. They reported some asymmetric overcrowded alkenes for chiroptical switches or motors [45, 84–87]. Take light-driven chiral motor 46 as example (Figure 6.26, top) [45, 87]. Its initial HTP at (P,P)-trans-form in nematic E7 is +99 μm−1, but generation of a cholesteric helix with an opposite sign of similar pitch is impossible, as the (M,M)-trans-form possesses a minor negative HTP (βM = −7 μm−1, E7). As a result of the high HTP at (P,P)-trans-form, colored LC films were easily generated using this dopant. Photochemical and thermal isomerization of the motor leads to irreversible color change in the LC film as shown in Figure 6.26 (bottom) [45].
Figure 6.26 Unidirectional rotation of molecular motor 46 in a liquid crystalline host, and associated helical twisting powers (top); colors of 46 doped LC phase (6.16 wt% in E7) in time, starting from pure (P,P)-trans-46 upon irradiation with >280 nm light at RT, as taken from actual photographs of the sample. The colors shown from left to right correspond to 0, 10, 20, 30, 40, and 80 s of irradiation time, respectively. Used with permission from Ref. [45].
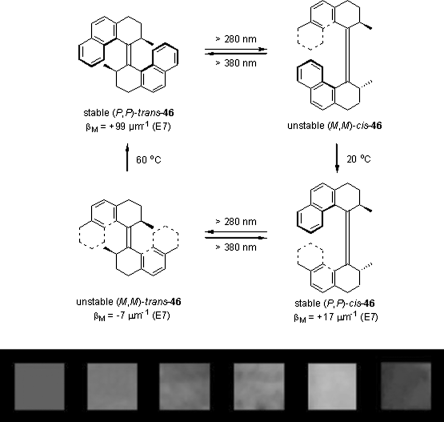
A breakthrough in this area was achieved with the introduction of fluorene-derived molecular motors. Possibly due to the structural compatibility of the fluorene group with the LC host's biphenyl core, motor 47 was found to possess very large helical twisting powers for both stable and unstable forms (Figure 6.27, top). Moreover, these two forms induce cholesteric phases of opposite signs, making it possible to switch efficiently between cholesteric helicities. As the thermal isomerization step (from unstable to stable form) occurs readily at room temperature, these motors were found to be able to induce fully reversible color change of a liquid crystalline film across the entire visible spectrum [88, 89]. Moreover, switching of this molecular motor in a liquid crystalline environment induced an unprecedented rotational reorganization of the LC film, which was applied in the light-driven rotation of microscale glass rods (Figure 6.27, bottom) [90, 91].
Figure 6.27 Features of a light-driven molecular motor: (a) Molecular structure of chiral motor 47. (b) Polygonal texture of a LC film doped with 1 wt% chiral motor 47. (c) Glass rod rotating on the LC during irradiation with ultraviolet light. Frames 1–4 (from left) were taken at 15-s intervals and show clockwise rotations of 28° (frame 2), 141° (frame 3), and 226° (frame 4) of the rod relative to the position in frame 1. Scale bars, 50 μm. (d) Surface structure of the LC film (atomic force microscopy image; 15 μm2). Used with permission from Ref. [90]. (See the color version of this figure in Color Plates section.)
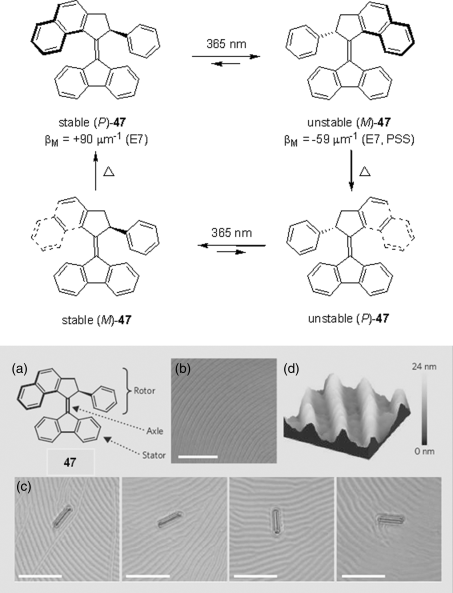
Besides, other groups also reported some chiral overcrowded alkenes as the dopants in LC media [92, 93]. Bunning et al. showed the polarized optical microscopy (POM) images of light-driven chiral motor 47 in nematic LC media (Figure 6.28). As shown in Figure 6.28a, the CLC consisted of 4.2 wt% 47 in LC1444 exhibiting a characteristic Grandjean texture expected of a short-pitch CLC. After exposure to 10 μW/cm2 UV light, the texture of the CLC remained in this state but undergoes color change, indicating a change in pitch. As the CLC pitch unwinds, a texture shown in Figure 6.28b was observed for the nematic phase. Continued UV exposure generates the fingerprint texture apparented in Figure 6.28c, characteristic of a long-pitch CLC. With continued UV exposure, the CLC again shows the Grandjean texture (Figure 6.28d–g). As evident in these panels, the number of defects in the Grandjean texture was initially low and then became larger. Continued light exposure seemed to annihilate some of these defects, as evident in Figure 6.28f and g. After UV exposure, POM images were also captured in the dark. As expected, the texture of the CLC evolves from Grandjean (Figure 6.28h) to nematic (Figure 6.28i) to fingerprint (Figure 6.28j) as the helix inverts.
Figure 6.28 POM images of 4.2 wt% 47 in LC1444 during exposure to 365 nm UV light (15 mW/cm2). The POM camera was filtered to 550 nm to avoid saturation with the UV light. (a) Grandjean texture before exposure (RCP, right-handed circularly polarized). (b) Formation of nematic during helical inversion. (c) Fingerprint texture after helical inversion. (d–g) Grandjean texture (LCP, left-handed circularly polarized) during UV exposure. (h) Defects disappear after UV light is removed. (i) Nematic phase during inversion. (j and k) Fingerprint texture after helical inversion. (l–n) Grandjean texture (RCP) restored in the dark. Used with permission from Ref. [93].
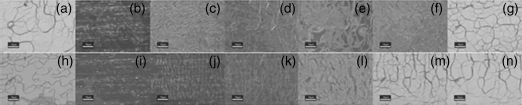
Furthermore, overcrowded alkenes have another pathway to show a switchable process in LC media which is caused by the chiral isomerization. This series of bistable switches of the overcrowded alkenes with an enantiomeric relationship between the two switch states can be interconverted by using circularly polarized light (CPL). It can be considered as a new type dopant, which exhibits the partial photoresolution under irradiating with CPL of one handedness. During the CPL process, the two enantiomers have different capability for absorbing the left-handed CPL (l-CPL) or right-handed CPL (r-CPL). As a result, one enantiomer is excited preferentially by either l-CPL or r-CPL within a racemic system, which will convert into the other enantiomer. However, this CPL being used has almost no effect to another enantiomer. On this occasion, the amount of the enantiomer will accumulate until an equilibrium or photostationary state (PSS) is reached. The enantiomeric excess (ee) value of this PSS (eePSS) at a certain wavelength of irradiation depends on the Kuhn anisotropy factor gλ, defined as the ratio of the circular dichroism (Δε) and the extinction coefficient (ε) (Eq. (6.1)) [94].
Normally, as g-value do not exceed 0.01, CPL photoresolution rarely leads to ee values over 0.5%. This ee values cannot be easily determined by the common methods. However, because the conversion from nematic to cholesteric is essentially thresholdless, theoretically these ee values are high enough to induce a nematic to cholesteric transition and can be determined from the cholesteric pitch via Equation (6.1). Similarly, the helicity of a cholesteric phase for this system can be controlled by only using the chiral information in the CPL. At last, the transition from cholesteric to nematic phase can be caused by irradiation with unpolarized light (UPL) or linearly polarized light (LPL), to lead to the racemization of chiral switch or motor [74].
Feringa et al. proved this concept by adopting the inherently dissymmetric overcrowded alkene 48 (Figure 6.29) [95]. They applied l-CPL irradiation at 313 nm to 20 wt% racemic 48 in a nematic LC K15 that can obtain the (M)-48 with 0.07% ee as a cholesteric phase. Then, irradiating the (M)-48 with LPL, the cholesteric LC phase gradually disappeared with the racemization. In the same way, the irradiation with r-CPL resulted in the cholesteric LC phase with opposite handedness, which still can go back to racemic state through LPL or UPL. Though the HTP (β) and the anisotropy factor (g) were both very low in this result, it did show the potential of this system for amplification of chirality via a chiral molecular switch to a macroscopic nematic to cholesteric phase transition by using a handedness CPL. In addition, this 3-stage LC switching system also presented how to control and develop between the positive and negative cholesteric LC phase.
Figure 6.29 CPL-induced deracemization of overcrowded alkene-based switch 48 in NLC resulting in 3-stage LC switching. PL, linearly polarized light; UPL, unpolarized light.

6.3.7 Axially Chiral Bicyclic Ketones as Dopants
Another series of reversible photoswitching of racemic bistable axially chiral bicyclic ketones irradiated by CPL, as mentioned previously in Section 3.6, was investigated by Schuster et al. [96–99]. Racemic axially chiral bicyclic ketone 49 was irradiated with l-CPL leading to the partial photoresolution (Figure 6.30) [96]. After irradiating for 6.7 h, a photostationary state was achieved with 0.4% ee, which is in good agreement with the calculated ee value from the anisotropy factor (g305 = 0.0105 at 305 nm). However, the enantiomeric enrichment cannot effectively cause the nematic to cholesteric phase transition, probably due to the low helical twisting power.
Figure 6.30 Deracemization of axially chiral bicyclic ketone 49 induced by CPL.
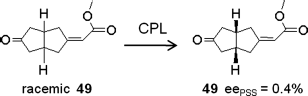
Several chiral bicyclic ketones 50–53 were designed as the photochemical molecular switches and applied as the triggers for the control of the LC phases (Figure 6.31) [96–99]. The structures of their rigid bicyclic core and ketone chromophore generally possess large g-values. Unfortunately, both the helical twisting power and solubility in nematic LC media are often low for most of them, which make it difficult to induce the nematic to cholesteric phase transition. Finally, they found the chiral bicyclic ketone 53 with a mesogenic unit, which resulted in a system capable of reversible nematic to cholesteric phase transition using the CPL resource (Figure 6.31) [99]. Ketone 53 contains a mesogenic moiety similar to the LC host ZLI-1167 resulting in a helical twisting power of 15 μm−1, a high g-value (g300 =0.016), and the good solubility. CPL irradiation (λ > 295 nm) of a nematic mixture containing 13 mol% racemic 53 resulted in a cholesteric phase with a pitch of 190 μm. This was more than twice the pitch obtained when a photo-resolved sample at the photostationary state was doped in the mesogenic host, probably due to scattering of the CPL by the LC mixture.
Figure 6.31 The examples of the chiral bicyclic ketones 50–53 designed by Schuster et al. and the process of nematic to cholesteric phase transition by CPL irradiation of the axially chiral bicyclic ketone 53.
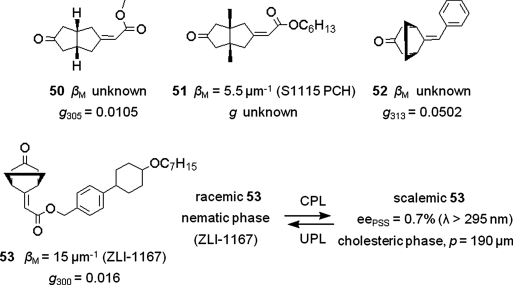
6.4 Conclusion
In this chapter, we have presented a brief overview about the dynamic behaviors and the properties of light-driven chiral molecular switches or motors in LC media. This kind of chiral molecular switches or motors doped into the LC media can be used as optical memory, optical display, and optical switching in the field of optical devices. As guest molecules, they can induce helical superstructures in an achiral LC host to obtain cholesteric LC and dynamically phototune the superstructures to achieve reversible reflection color or/and handedness inversion etc. Moreover, the phenomenon of cholesteric induction is a remarkable example of how the chiral information at the molecular level can be transmitted through amplification in self-organized stimuli-responsive soft matter. From the above discussions, it is clear that adding small quantities of chiral dopants to achiral liquid crystals have become the method of choice for helicity induction in liquid crystals. Furthermore, liquid crystals can serve as model systems in the development of supramolecular assemblies with controlled chiral architectures induced by stimuli-responsive chiral triggers.
The continuous efforts on finding new efficient photoswitchable and soluble chiral dopants are expected to provide better understanding of chiral induction in soft matter and could provide future smart materials and devices with improved properties and performance. Although the calamitic nematic phase has been largely exploited in this endeavor, the nematic phases exhibited by discotic and bent-core liquid crystals are still left to be explored. Finally the development of novel switchable chiral dopants with very high HTP in very small quantities as low as parts per million (ppm) and which can aid fast and reversible phototuning of reflection colors over the entire visible spectrum is urgently required to fully explore the potential of these intriguing materials. Open research fields also include other LC phases with induced chirality, like blue phases and smectic C* phases, as well as chiral doped micelles.
Acknowledgments
The preparation of this chapter benefited from the support to Quan Li by the Ohio Board of Regents under its Research Challenge program, the Air Force Office of Scientific Research (AFOSR FA9950-09-1-0193 and FA9950-09-1-0254), the Department of Energy (DOE DE-SC0001412), the Department of Defense Multidisciplinary University Research Initiative (AFOSR MURI FA 9550-06-1-0337 and FA9550-12-1-0037), and the National Science Foundation (NSF IIP 0750379).
1. S. Kinoshita and S. Yoshioka. Structural colors in nature: the role of regularity and irregularity in the structure. Chem Phys Chem 2005, 6, 1442–1459.
2. S. Bertheier. Iridescences: The Physical Color of Insects, Springer, New York, 2007.
3. D. Graham-Rowe. Tunable structural color. Nat. Photonics 2009, 3, 551–553.
4. V. Sharma, M. Crne, J. Park, and M. Srinivasarao. Structural origin of circularly polarized iridescence in jeweled beetles. Science 2009, 325, 449–451.
5. B. L. Feringa. The art of building small: from molecular switches to molecular motors. J. Org. Chem. 2007, 72, 6635–6652.
6. K. Ichimura.In: H. Dürr and H. Bouas-Laurent, Eds., Photochromism: Molecules and Systems, Elsevier, Amsterdam, 1990.
7. M. Mathews, R. Zola, D. Yang, and Q. Li. Thermally, photochemically and electrically switchable reflection colors from self-organized chiral bent-core liquid crystals. J. Mater. Chem. 2011, 21, 2098–2103.
8. G. Heppke and F. Oestreicher. Bestimmung des helixdrehsinns cholesterischer phasen mit der Grandjean-Cano-methode. Z. Naturforsch. 1977, 32, 899–901.
9. G. Heppke and F. Oestreicher. Determination of the cholesteric screw sense. Mol. Cryst. Liq. Lett. 1978, 41, 245–249.
10. P. R. Gerber. On the determination of the cholesteric screw sense by the Grandjean–Cano-method. Z. Naturforsch. 1980, 35, 619–622.
11. I. Dierking. Textures of Liquid Crystals, Wiley-VCH, Weinheim, 2003.
12. G. Solladié and R. G. Zimmermann. Liquid crystals: a tool for studies on chirality. Angew. Chem. Int. Ed. 1984, 23, 348–362.
13. G. P. Spada and G. Proni. The nematic liquid crystal phase as a probe of the molecular shape helicity. Enantiomer 1998, 3, 301–314.
14. G. Proni and G. P. Spada. Doped nematic phases: a tool for amplifying and detecting chirality. Enantiomer 2001, 6, 171–179.
15. B. L. Feringa. Molecular Switches, Wiley–VCH, Germany, 2001.
16. N. Tamaoki. Cholesteric liquid crystals for color information technology. Adv. Mater. 2001, 13, 1135–1147.
17. S. Pieraccini, S. Masiero, A. Ferrarini, and G. P. Spada. Chirality transfer across length-scales in nematic liquid crystals: fundamentals and applications. Chem. Soc. Rev. 2011, 40, 258–271.
18. T. Ikeda. Photomodulation of liquid crystal orientations for photonic applications. J. Mater. Chem. 2003, 13, 2037–2057.
19. V. Balzani, A. Credi, F. M. Raymo, and J. F. Stoddart. Artificial molecular machines. Angew. Chem. Int. Ed. 2000, 39, 3348–3391.
20. The May 2000 issue of Chem. Rev.: Memories and Switches.
21. F. L. Carter, H. Siatkowski, and H. Wohltgen. Molecular Electronic Devices, Elsevier, Amsterdam, 1988.
22. E. Sackmann. Photochemically induced reversible color changes in cholesteric liquid crystals. J. Am. Chem. Soc. 1971, 93, 7088–7090.
23. D. Pijper and B. L. Feringa. Control of dynamic helicity at the macro- and supramolecular level. Soft Matter 2008, 4, 1349–1372.
24. K. Ichimura. Photoalignment of liquid-crystal systems. Chem. Rev. 2000, 100, 1847–1873.
25. T. Ikeda. Photomodulation of liquid crystal orientations for photonic applications. J. Mater. Chem. 2003, 13, 2037–2057.
26. M. Moriyama, S. Song, H. Matsuda, and N. Tamaoki. Effects of doped dialkylazobenzenes on helical pitch of cholesteric liquid crystal with medium molecular weight: utilisation for full-colour image recording. J. Mater. Chem. 2001, 11, 1003–1010.
27. S. Kurihara, T. Kanda, T. Nagase, and T. Nonaka. Photochemical color switching behavior of induced cholesteric liquid crystals for polarizer free liquid crystalline devices. Appl. Phys. Lett. 1998, 73, 2081–2083.
28. N. Tamaoki, S. Song, M. Moriyama, and H. Matsuda. Rewritable full-color recording in a photon mode. Adv. Mater. 2000, 12, 94–97.
29. S. V. Serak, E. O. Arikainen, H. F. Gleeson, V. A. Grozhik, J.-P. Guillou, and N. A. Usova. Laser-induced concentric colour domains in a cholesteric liquid crystal mixture containing a nematic azobenzene dopant. Liq. Cryst. 2002, 29, 19–26.
30. S. Tazuke, S. Kurihara, and T. Ikeda. Amplified image recording in liquid crystal. Media by means of photochemically triggered phase transition. Chem. Lett. 1987, 911–914.
31. C. Ruslim and K. Ichimura. Conformational effect on macroscopic chirality modification of cholesteric mesophases by photochromic azobenzene dopants. J. Phys. Chem. B 2000, 104, 6529–6535.
32. C. Ruslim, M. Nakagawa, S. Morino, and K. Ichimura. Chiroptical properties of cholesteric liquid crystals induced by chiral photochromic 3,3′-dialkoxyazobenzenes. Mol. Cryst. Liq. Cryst. 2001, 365, 55–62.
33. T. Yoshioka, M. D. Z. Alam, T. Ogata, T. Nonaka, and S. Kurihara. Photochemical tuning of the helical structure of cholesteric liquid crystals by photoisomerization of chiral azobenzenes, and their structural effects. Liq. Cryst. 2004, 31, 1285–1291.
34. S. Kurihara, S. Nomiyama, and T. Nonaka. Photochemical switching between a compensated nematic phase and a twisted nematic phase by photoisomerization of chiral azobenzene molecules. Chem. Mater. 2000, 12, 9–12.
35. S. Kurihara, S. Nomiyama, and T. Nonaka. Photochemical control of the macrostructure of cholesteric liquid crystals by means of photoisomerization of chiral azobenzene molecules. Chem. Mater. 2001, 13, 1992–1997.
36. S. Pieraccini, G. Gottarelli, R. Labruto, S. Masiero, O. Pandoli, and G. P. Spada. The control of the cholesteric pitch by some azo photochemical chiral switches. Chem. Eur. J. 2004, 10, 5632–5639.
37. S. Pieraccini, S. Masiero, G. P. Spada, and G. Gottarelli. A new axially-chiral photochemical switch. Chem. Commun. 2003, 598–599.
38. R. A. van Delden, T. Mecca, C. Rosini, and B. L. Feringa. A chiroptical molecular switch with distinct chiral and photochromic entities and its application in optical switching of a cholesteric liquid crystal. Chem. Eur. J. 2004, 10, 61–70.
39. J. Ma, Y. Li, T. White, A. Urbas, and Q. Li. Light-driven nanoscale chiral molecular switch: reversible dynamic full range color phototuning. Chem. Commun. 2010, 46, 3463–3465.
40. Q. Li, L. Li, J. Kim, H.-K. Park, and J. Williams. Reversible photoresponsive chiral liquid crystals containing cholesteryl moiety and azobenzene linker. Chem. Mater. 2005, 17, 6018–6021.
41. C. Ruslim and K. Ichimura. Conformation-assisted amplification of chirality transfer of chiral Z-azobenzenes. Adv. Mater. 2001, 13, 37–40.
42. C. Ruslim and K. Ichimura. Photoswitching in chiral nematic liquid crystals: interaction-selective helical twist inversion by a single chiral dopant. J. Mater. Chem. 2002, 12, 3377–3379.
43. T. Yoshioka, T. Ogata, T. Nonaka, M. Moritsugu, S.-N. Kim, and S. Kurihara. Reversible-photon-mode full-color display by means of photochemical modulation of a helically cholesteric structure. Adv. Mater. 2005, 17, 1226–1229.
44. R. A. van Delden, M. B. van Gelder, N. P. M. Huc, and B. L. Feringa. Controlling the color of cholesteric liquid-crystalline films by photoirradiation of a chiroptical molecular switch used as dopant. Adv. Funct. Mater. 2003, 13, 319–324.
45. R. A. van Delden, N. Koumura, N. Harada, and B. L. Feringa. Unidirectional rotary motion in a liquid crystalline environment: color tuning by a molecular motor. Proc. Natl. Acad. Sci. U.S.A. 2002, 99, 4945–4949.
46. Q. Li, L. Green, N. Venkataraman, I. Shiyanovskaya, A. Khan, A. Urbas, and J. W. Doane. Reversible photoswitchable axially chiral dopants with high helical twisting power. J. Am. Chem. Soc. 2007, 129, 12908–12909.
47. E. Montbath, N. Venkataraman, A. Khan, I. Shiyanovskaya, T. Schneider, J. W. Doane, L. Green, and Q. Li. Novel optically addressable photochiral displays. SID Digest Tech. Pap. 2008, 919–922.
48. N. Venkataraman, G. Magyar, E. Montbath, A. Khan, T. Schneider, J. W. Doane, L. Green, and Q. Li. Thin flexible photosensitive cholesteric displays. J. Soc. Information Display 2009, 17, 869–873.
49. D.-K. Yang and J. W. Doane. Cholesteric liquid crystal/polymer gel dispersions: reflective display application. SID Int. Symp. Digest Tech. Papers 1992, 23, 759–761.
50. D.-K. Yang, J. L. West, L.-C. Chien, and J. W. Doane. Control of reflectivity and bistability in displays using cholesteric liquid crystals. J. Appl. Phys. 1994, 76, 1331–1333.
51. T. J. White, R. L. Bricker, L. V. Natarajan, N. V. Tabiryan, L. Green, Q. Li, and T. J. Bunning, Phototunable azobenzene cholesteric liquid crystals with 2000 nm range. Adv. Funct. Mater. 2009, 19, 3484–3488.
52. L. Green, Y. Li, T. White, A. Urbas, T. Bunning, and Q. Li. Light-driven molecular switches with tetrahedral and axial chirality. Org. Biomol. Chem. 2009, 7, 3930–3933.
53. J. Chen, S. M. Morris, T. D. Wilkinson, and H. J. Coles. Reversible color switching from blue to red in a polymer stabilized chiral nematic liquid crystals. Appl. Phys. Lett. 2007, 91, 121118.
54. M. Kawamoto, T. Aoki, and T. Wada. Light-driven twisting behaviour of chiral cyclic compounds. Chem. Commun. 2007, 930–932.
55. M. Mathews and N. Tamaoki. Planar chiral azobenzenophanes as chiroptic switches for photon mode reversible reflection color control in induced chiral nematic liquid crystals. J. Am. Chem. Soc. 2008, 130, 11409–11416.
56. M. Mathews, R. S. Zola, S. Hurley, D.-K. Yang, T. J. White, T. J. Bunning, and Q. Li. Light-driven reversible handedness inversion in self-organized helical superstructures. J. Am. Chem. Soc. 2010, 132, 18361–18366.
57. M. Kawamoto, N. Shiga, K. Takashi, and T. Yamashita. Non-destructive erasable molecular switches and memory using light-driven twisting motions. Chem. Commun. 2010, 46, 8344–8346.
58. P. M. A. Bonaccorsi, D. A. Dunmur, and J. F. Stoddart. Cholesteric phases induced by chiral substituted cyclohexylidenemethanes. New J. Chem. 1988, 12, 83–85.
59. S. N. Yarmolenko, L. A. Kutulya, V. V. Vashchenko, and L. V. Chepeleva. Photosensitive chiral dopants with high twisting power. Liq. Cryst. 1994, 16, 877–882.
60. E. Mena, P. V. D. Witte, and J. Lub. Camphor and nopinone derivatives as new photosensitive chiral dopants. Liq. Cryst. 2000, 27, 929–933.
61. P. V. D. Witte, J. C. Galan, and J. Lub. Modification of the pitch of chiral nematic liquid crystals by means of photoisomerization of chiral dopants. Liq. Cryst. 1998, 24, 819–827.
62. J. Lub, A. Ferrer, C. Larossa, and B. Malo. Synthesis and properties of chiral stilbene diacrylates. Liq. Cryst. 2003, 30, 1207–1218.
63. J. Lub, W. P. M. Nijssen, R. T. Wegh, I. De Francisco, M. P. Ezquerro, and B. Malo. Photoisomerizable chiral compounds derived from isoserbide and cinnamic acid. Liq. Cryst. 2005, 32, 1031–1044.
64. J. Lub, W. P. M. Nijssen, R. T. Wegh, J. P. A. Vogels, and A. Ferrer. Synthesis and properties of photoisomerizable derivatives of isosorbide and their use in cholesteric filters. Adv. Funct. Mater. 2005, 15, 1961–1972.
65. M. Irie. Diarylethenes for memories and switches. Chem. Rev. 2000, 100, 1685–1716.
66. C. Denekamp and B. L. Feringa. Optically active diarylethenes for multimode photoswitching between liquid-crystalline phases. Adv. Mater. 1998, 10, 1080–1082.
67. T. van Leeuwen, T. C. Pijper, J. Areephong, B. L. Feringa, W. R. Browne, and N. Katsonis. Reversible photochemical control of cholesteric liquid crystals with a diamine-based diarylethene chiroptical switch. J. Mater. Chem. 2011, 21, 3142–3146.
68. T. Yamaguchi, H. Nakazumi, K. Uchida, and M. Irie. Photochromism of a chiral cyclohexane having two diarylethene chromophores. A large optical rotation change. Chem. Lett. 1999, 653–654.
69. T. Yamaguchi, T. Inagawa, H. Nakazumi, S. Irie, and M. Irie. Photoswitching of helical twisting power of a chiral diarylethene dopant: pitch change in a chiral nematic liquid crystal. Chem. Mater. 2000, 12, 869–871.
70. T. Yamaguchi, T. Inagawa, H. Nakazumi, S. Irie, and M. Irie. Photoswitching of helical twisting power by chiral diarylethene dopants. Mol. Cryst. Liq. Cryst. 2001, 365, 861–866.
71. T. Yamaguchi, T. Inagawa, H. Nakazumi, S. Irie, and M. Irie. Phase transition of a liquid induced by chiral photochromic dopants. Mol. Cryst. Liq. Cryst. 2000, 345, 287–292.
72. T. Yamaguchi, T. Inagawa, H. Nakazumi, S. Irie, and M. Irie. Photoinduced pitch changes in chiral nematic liquid crystals formed by doping with chiral diarylethene. J. Mater. Chem. 2001, 11, 2453–2458.
73. T. van Leeuwen, T. C. Pijper, J. Areephong, B. L. Feringa, W. R. Browne, and N. Katsonis. Reversible photochemical control of cholesteric liquid crystals with a diamine-based diarylethene chiroptical switch. J. Mater. Chem. 2011, 21, 3142–3146.
74. R. Eelkema and B. L. Feringa. Amplification of chirality in liquid crystals. Org. Biomol. Chem. 2006, 4, 3729–3745.
75. K. Rameshbabu, A. Urbas, and Q. Li. Synthesis and characterization of thermally irreversible photochromic cholesteric liquid crystals. J. Phys. Chem. B 2011, 115, 3409–3415.
76. Y. Li, A. Urbas, and Q. Li. Synthesis and characterization of light-driven dithienylcyclopentene switches with axial chirality. J. Org. Chem. 2011, doi: 10.1021/jo201139t.
77. B. L. Feringa, R. A. van Delden, N. Koumura, and E. M. Geertsema. Chiroptical molecular switches. Chem. Rev. 2000, 100, 1789–1816.
78. H. Hattori and T. Uryu. Photochromic chiral liquid crystalline systems containing spiro-oxazine with a chiral substituent II. Photoinduced behavior. Liq. Cryst. 2001, 28, 1099–1104.
79. L.-M. Jin, Y. Li, J. Ma, and Q. Li. Synthesis of novel thermally reversible photochromic axially chiral spirooxazines. Org. Lett. 2010, 12, 3552–3555.
80. Y. Yokoyama. Fulgides for memories and switches. Chem. Rev. 2000, 100, 1717–1739.
81. Y. Yokoyama and T. Sagisaka. Reversible control of pitch of induced cholesteric liquid crystal by optically active photochromic fulgide derivatives. Chem. Lett. 1997, 687–688.
82. Y. Yokoyama, S. Uchida, Y. Yokoyama, T. Sagisaka, Y. Uchida, and T. Inada. Chiral photochromic compounds and control of functions. Enantiomer 1998, 3, 123–132.
83. T. Sagisaka and Y. Yokoyama. Reversible control of the pitch of cholesteric liquid crystals by photochromism of chiral fulgide derivatives. Bull. Chem. Soc. Jpn. 2000, 73, 191–196.
84. B. L. Feringa and H. Wynberg. Torsionally distorted olefins. Resolution of cis- and trans-4,4′-bi-1,1′,2,2′,3,3′,-hexahydrophenanthrylidene. J. Am. Chem. Soc. 1977, 602–603.
85. B. L. Feringa, N. P. M. Huck, and H. A. V. Doren. Chiroptical switching between liquid crystalline phases. J. Am. Chem. Soc. 1995, 117, 9929–9930.
86. B. L. Feringa, W. F. Jager, B. De Lange, and E. W. Meijer. Chiroptical molecular switch. J. Am. Chem. Soc. 1991, 113, 5468–5470.
87. N. Koumura, R. W. J. Zijlstra, R. A. van Delden, N. Harada, and B. L. Feringa. Light-driven monodirectional molecular rotor. Nature 1999, 401, 152–155.
88. R. Eelkema and B. L. Feringa. Reversible full-range color control of a cholesteric liquid-crystalline film by using a molecular motor. Chem. Asian J. 2006, 1, 367–369.
89. A. Bosco, M. G. M. Jongejan, R. Eelkema, N. Katsonis, E. Lacaze, A. Ferrarini, and B. L. Feringa. Photoinduced reorganization of motor-doped chiral liquid crystals: bridging molecular isomerization and texture rotation. J. Am. Chem. Soc. 2008, 130, 14615–14624.
90. R. Eelkema, M. M. Pollard, J. Vicario, N. Katsonis, B. Serrano Ramon, C. W. M. Bastiaansen, D. J. Broer, and B. L. Feringa. Molecular machines: nanomotor rotates microscale objects. Nature 2006, 440, 163.
91. R. Eelkema, M. M. Pollard, N. Katsonis, J. Vicario, D. J. Broer, and B. L. Feringa. Rotational reorganization of doped cholesteric liquid crystalline films. J. Am. Chem. Soc. 2006, 128, 14397–14407.
92. C.-T. Chen and Y.-C. Chou. C2-symmetric dibenzosuberane-based helicenes as potential chirochromic optical switches. J. Am. Chem. Soc. 2000, 122, 7662–7672.
93. T. J. White, S. A. Cazzell, A. S. Freer, D.-K. Yang, L. Sukhomlinova, L. Su, T. Kosa, B. Taheri, and T. J. Bunning. Widely tunable, photoinvertible cholesteric liquid crystals. Adv. Mater. 2011, 23, 1389–1392.
94. K. L. Stevenson and J. F. Verdieck. Photodestruction and photoresolution with CPL has been achieved. J. Am. Chem. Soc. 1968, 90, 2974–2975.
95. N. P. M. Huck, W. F. Jager, B. de Lange, and B. L. Feringa. Dynamic control and amplification of molecular chirality by circular polarized light. Science 1996, 273, 1686–1688.
96. M. Suarez and G. B. Schuster. Photoresolution of an axially chiral bicyclo [3.3.0]octan-3-one: phototriggers for a liquid crystal-based optical switch. J. Am. Chem. Soc. 1995, 117, 6732–6738.
97. Y. Zhang and G. B. Schuster. Chirochromism–photochromism by epimerization: search for a liquid crystal phototrigger. J. Am. Chem. Soc. 1994, 116, 4852–4857.
98. Y. Zhang and G. B. Schuster. Photoresolution of an axially chiral bicyclo [3.2.1]octan-3-one: phototriggers for a liquid crystal-based optical switch. J. Org. Chem. 1995, 60, 7192–7197.
99. K. S. Burnham and G. B. Schuster. Transfer of chirality from circularly polarized light to a bulk material property: propagation of photoresolution by a liquid crystal transition. J. Am. Chem. Soc. 1999, 121, 10245–10246.