Chapter 2
Self-Organized Semiconducting Discotic Liquid Crystals for Optoelectronic Applications
2.1 Introduction
Why are almost all of the leaves on earth green? Because they have a superior light harvesting material, i.e., chlorophyll, to efficiently absorb sunlight for photochemical reactions. This material evolved to be the best for the above purpose since it was produced after hundreds of millions of years' natural selection. Interestingly, the key component of chlorophyll is porphyrin, a disc-like aromatic molecule with a large π-conjugated system. In many cases, when large π-conjugated aromatic cores are linked with flexible aliphatic peripheral substituents, a discotic liquid crystalline (DLC) phase may appear. Since the work of Chandrasekhar on the hexaesters of benzene published in 1977 [1], DLC materials have been investigated intensively, especially over the last decade. Successful commercialization of DLC materials has been accomplished in Fuji “Wide-View” (WV) optical compensation films [2, 3]. Apart from the use in display, they have been widely and deeply investigated starting from structure–properties to structure–device performance relationships.
In recent decades, there has been increasing attention in the research field of organic electronics for device applications such as photovoltaic devices (PVD), light-emitting-diodes (LEDs), field-effect transistors (FETs), memory elements, and sensors. Particularly in the area of developing organic photovoltaic devices for reducing the demand of fossil fuels in the world, this research has been expected to create cheap, flexible thin films to replace the expensive and not easily processable inorganic materials. Among the diverse new materials for organic semiconductors, conjugated liquid crystals (LCs) hold a particular promise due to their extraordinary abilities of self-organization and self-healing that can remove structural defects, such as grain boundaries, which are detrimental to charge transport. By thermal annealing, the spontaneous formation of large single domains could be achieved [4, 5]. Among these conjugated LCs, discotic molecules are well known for their disc-like molecular shape, different from their rod-like (calamitic) counterparts as shown in Figure 2.1 [6]. Owing to this particular type of molecular shape, quite different phase symmetry, direction of charge transport, and extent of π-orbital overlap have been observed. For discotic molecules, there is more than one assembling state in LC phase depending on the type of lattice (Figure 2.2) [7]. The least ordered mesophase formed by disc-like molecules is the nematic (ND) phase (a). The ND phase is analogous to the nematic phase formed by rod-like molecules and is characterized by the presence of only orientational order where the mesogens align with their principal axes along one direction. More common phases exhibited by disc-like molecules are columnar phases, in which the disc-like molecules stack into columns. Columnar phases show a rich poly-mesomorphism, which are generally classified according to their symmetry, the degree of ordering within the column, and the orientation of the discs with respect to the columnar axis [8, 9]. Apart from the columnar nematic phase (b) showing different directors of orientational order of columns, other columnar phases show identical orientational order plus positional order. Among them, a hexagonal columnar (Colh) phase (c) and a rectangular columnar (Colr) phase (d) with tilted columns are the two most common types of columnar phases. However, more ordered phases such as columnar oblique (Colob) (e), columnar plastic (Colp) (f), columnar helical (g), and columnar lamellar (h) mesophases are not as common. For the same molecule, when temperature changes they may transfer from one mesophase to another with variant ordered states. For example, one can make a distinction between the least ordered ND phase, Colh phase, and Colr phase when temperature decreases as shown in Figure 2.3. These different mesophases can be experimentally characterized by the combined use of techniques. For textural observation, we can use polarizing optical microscopy (POM); for thermal analysis, we can use differential scanning calorimetry (DSC); and for molecular packing structures determination, we can use X-ray diffraction (XRD).
Figure 2.1 Schematic drawing of some prototype calamitic (left) and discotic (right) liquid crystal molecules.

Figure 2.2 Schematic drawings of common mesophases of disc-shaped molecules: (a) discotic nematic, (b) columnar nematic, (c) hexagonal columnar, (d) rectangular columnar, (e) columnar oblique, (f) columnar plastic, (g) columnar helical, and (h) columnar lamellar phase.
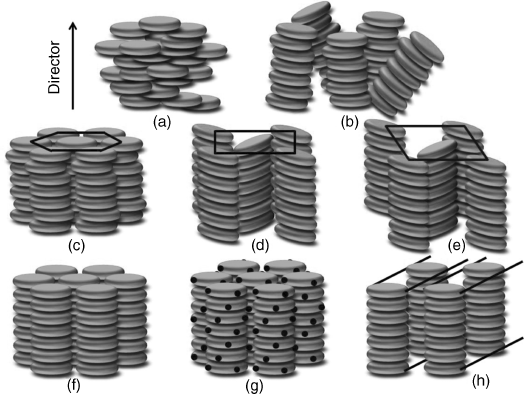
Figure 2.3 The phases formed by disc-like molecules are distinguished by the degree of positional order and symmetry, and they may be rearranged from one mesophase to another upon temperature change.
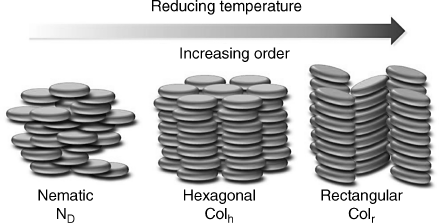
The particular two-dimensional chemical structure of discotic molecules brings new features. First of all, within columns the adjacent disc-like molecules have a much larger orbital overlap than calamitics. As a result, the band width reaches a value as high as 1.1 eV, close to that of graphite (ca. 1.0–1.4 eV) [10]. Secondly, the large orbital overlap between stacked disc-like molecules results in high values of the charge carrier mobility (μ) in their liquid crystalline mesophases, which can be 0.2–1.3 cm2 V−1 s−1 [11–14]. Thirdly, in the discotic columnar mesophase, the exciton diffusion length in discotics is able to exceed 70 nm [15], which is much higher than that of most conventional conjugated polymers (ca. 10–20 nm).
In this chapter, progress in the research field of DLC materials is comprehensively outlined. First, charge transport measurements of DLCs are briefly introduced. Second, molecular structures of most typical DLCs and novel systems are presented. Third, as the supramolecular alignment of DLC is critically controlled by the processing methods, important techniques are described. In the last part, a general introduction of most important optoelectronic applications of DLCs are demonstrated.
2.2 Charge Transport and Measurements in DLCS
2.2.1 Charge Transport in DLC Semiconductors
For organic semiconducting materials in a conjugated system, π-orbital wave functions of adjacent carbon atoms overlap and the electrons occupying such orbitals become relatively delocalized. Figure 2.4 clearly shows this fundamental but essential molecular electronic feature. Each conjugated carbon atom in the sp2 hybridization of a molecule forms 3 σ-bonds from the overlapping of hybridized 2s, 2px and 2py valence atomic orbitals; the fourth 2pz orbital is perpendicular to the σ-bond plane and laterally overlaps to form the π-bonds. σ-Bond is strong and the energy difference between the occupied bonding orbital (σ) and the unoccupied antibonding orbital (σ*) is quite large. π-Bond is weaker than σ-bond, and it has a much smaller energy difference between the highest occupied molecular π-orbital (HOMO) and the lowest unoccupied molecular orbital (LUMO). The corresponding lower band gap energy determines the semiconducting properties of a molecule. For semiconducting materials, the forming energy of band gaps is in the range of 1.5–3 eV.
Figure 2.4 (a) Schematic illustration of σ-bond and π-bond formation between two sp2 hybridized carbons. (b) An orbital energy-level diagram for the C–C bond formation between two sp2 hybridized carbons. (c) Schematic representation of the organization of discotic molecules into an one-dimensional conducting column.
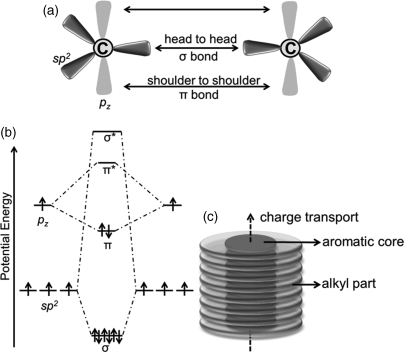
Compared with inorganic semiconductors, organic semiconductors are cheap, easy to process, excellent for casting large films, and capable of being deposited on flexible substrates such as plastics. Although it is generally recognized that the charge transport mobility is not as high as inorganic semiconductors, great efforts are being made and the mobilities are catching up.
Electron and hole transport in organic semiconductors occurs via π–π coupling between the HOMO and LUMO orbitals respectively of neighboring sites. In discotic columnar mesophases, the charge carrier mobility was relatively high since the cores of the conjugated aromatic systems self-organize into long-range ordered, π-orbital overlapped columnar structures (inter-core distances of about 3.5 Å), providing pathways to facilitate charge carrier transport between adjacent molecules (in Figure 2.4c). Generally speaking, charge-carrier mobility in such discotic columns can reach from 10−3 to 1.0 cm2 V−1 s−1. For the charge mobilities less than 0.1 cm2 V−1 s−1 in DLC semiconductors, a one-dimensional hopping process was postulated [16, 17]. For the carrier mobilities more than 0.1 cm2 V−1 s−1, a band-like charge transport that involves the formation of conduction bands across several molecules was proposed [18]. Discotic columns can be considered as one-dimensional conducting molecular wires/belt because of the anisotropic conducting behavior in the columnar mesophase, in which the charges transport along the aromatic cores. Furthermore, the peripheral chains (usually flexible alkyls) linking to the rigid core form an insulating hydrocarbon matrix prohibiting charge carriers from transporting between columns. Depending on the ionization potential or electron affinity, the discotic molecules can transport either positive holes or negative electrons or both under certain conditions [19]. Based on the charge transport property, such DLC semiconducting materials can be classified as three types: p-type, n-type or ambipolar.
2.2.2 Measurements of Charge Mobility
The charge carrier mobility is a key parameter in determining the performance of a semiconducting material. In the past decades, various techniques have been developed to determine the charge mobility value and the most widely used ones are briefly described below.
2.2.2.1 Time of Flight (TOF)
Charge mobilities in organic materials were first measured with the TOF technique by Kepler [20] and Leblanc [21]. In this technique as shown in Figure 2.5, one organic layer of a few micrometers thick is sandwiched between two electrodes with known inter-distance. Charges are generated by irradiating a laser pulse with energy greater than or equal to the band gap towards materials in the proximity of one electrode. The generated holes or electrons migrate across the organic material layer to the second electrode under an applied electric field depending on the polarity of the applied bias and the corresponding electric field (in the range of 104–106 V cm−1). The current at that electrode is recorded as a function of time, which enables one to study the mobility by measuring the transient time [22]. The mobility (μ) of the holes or electrons is estimated as:
Figure 2.5 Schematic representation of the TOF experiment.
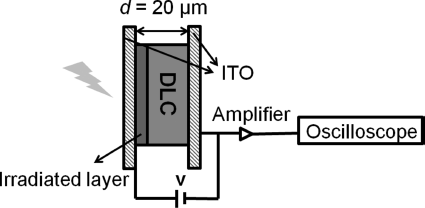
where ν is the velocity, F is the electric field, d is the distance between the electrodes, t is the averaged transient time, and V is the applied voltage.
Generally, a sharp signal is obtained in the case of ordered materials while in disordered systems a broader signal occurs because of the distribution of transient times for charges passing across the material. This is mostly due to shallow trapping often caused by structural defects in the materials which cause variations of mobilities. It has been revealed that the mobility decreases with the reduction of molecular order from crystal, liquid crystal to isotropic phase [23].
Measurements of the TOF technique for DLCs exhibit values usually on the order of 10−3 cm2 V−1 s−1, while a value as high as 0.2 cm2 V−1 s−1 has also been reported [24]. Because imperfections in the columnar arrangement can have a negative influence on charge carrier transport and a lower limit value will show up, self-organized molecules possessing a degree of order as high as possible within the inter-electrode gap are desired.
2.2.2.2 Pulse-Radiolysis Time-Resolved Microwave Conductivity (PR-TRMC)
In the PR-TRMC technique, the sample (bulk material) is first excited by a pulse of highly energetic electrons (in the MeV range) to create a low density of free charge carriers. As shown in Figure 2.6, charge carriers are created by using nanosecond-duration pulses of ionizing radiation from a Van de Graaff accelerator [25]. New generated charge carriers, induced by the pulse, will increase the conductivity of the sample, then a frequency dependent deviation of the microwave power can be detected. The change of conductivity Δσ is expressed as [26]:
Figure 2.6 Schematic illustration of the PR-TRMC experimental setup.
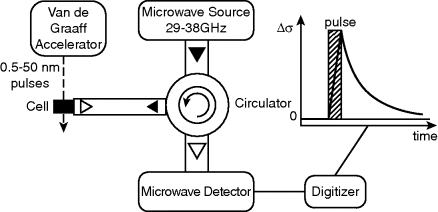
where Σμ is the sum of hole and electron mobilities and Ne–h is the density of generated electron–hole pairs. From PR-TRMC measurements, the sum of positive and negative charge-carrier mobilities: Σμ = μ(+) + μ(−) is yielded and it is not possible to separate the positive and negative carriers. This Ne–h value is calculated as follows: a ratio of the amount of energy density transferred to the material by the energy required to create one electron–hole pair is first obtained, then this ratio is further multiplied by a survival probability because during the standing of the pulse there is possible charge recombination.
PR-TRMC is a contact-free technique which is not affected by space-charge effects. It requires no electrode deposition onto the sample and the interference of the electrode can be excluded. Moreover, the charges are directly generated in the bulk and the charges trapped by structural defects or impurities are not responsive. Therefore, the mobility of intrinsic charge carriers can be determined even for multi-domain samples without alignment. Because PR-TRMC measurement of the mobility shows local-wise character which is over a short range (a few molecules) and on a nanosecond timescale, the resulting intrinsic AC mobility value for the bulk is considered to be the upper limit for a sample at low field. On the contrary, in TOF technique the DC measurements probing a macroscopic range are generally smaller since the charge carriers are crossing structural defects and interacting with impurities. Thus, depending on the degree of order in the sample, AC and DC mobility values deviate above a threshold frequency. A class of DLC materials hexahexylthiotriphenylene (HHTT) have been used as reference compounds to validate the PR-TRMC technique [27], and with this technique, mobility values as high as 1.1 cm2 V−1 s−1 have been achieved in a discotic hexabenzocoronene (HBC) derivative [28].
2.2.2.3 Space-Charge-Limited Current (SCLC)
The charge mobility behavior can be characterized by the SCLC technique, in which an organic layer is sandwiched between two electrodes. The charge transport is bulk dependent but not contact dependent. Ohmic contacts are assumed. In this technique, when the organic layer's intrinsic electrostatic potential equals that of the electrode, the injection of additional charges can be prevented, the current density is closest to that of the injecting electrode, and the obtained mobility value is the maximum [29, 30]. In this technique, when neglecting diffusion contributions, the current density J can be expressed as:
where denotes the dielectric constant of the organic material, V is the applied bias voltage, and L is the device thickness. At high electric fields, the field-dependence of the mobility has to be considered. Generally, the electrodes are chosen based on when electrons or holes can be injected at low voltage.
Normally when traps are present, the J–V curve becomes complex, exhibiting a linear regime where transport is injection-limited. Then a sudden increase for an intermediate range of applied biases appears, and at last the V2-dependence of the trap-free SCLC regime is reached.
2.2.2.4 FET
Electrical characteristics measurement in a FET configuration can also provide the carrier mobilities. Horowitz has pointed out that the I–V (current–voltage) expressions derived for inorganic transistors in the linear and saturated regimes are ready to be applied to organic field-effect transistors (OFETs) [31]. The expressions are listed below:
in the linear regime,
in the saturated regime,
ISD and VSD are the current and voltage bias between source and drain, VG is the gate voltage, VT is the threshold voltage at which the current starts to rise, C is the capacitance of the gate dielectric, W and L are the width and length of the conducting channel respectively, and μ is the carrier mobility.
In FETs, the charges transport within a very narrow channel (no more than a few nanometers wide) at the interface between the organic semiconductor and the dielectric [32, 33]. There are many factors influencing the charge transport, for example, the structural defects in the organic layer at the interface, the surface topology and polarity of the dielectric, the presence of defects at the interface depending on the structure of the gate dielectric surface materials, and contact resistance at the source and drain metal/organic interfaces [34–36]. The dielectric constant of the gate insulator also affects the mobility; the carrier mobility decreases with increasing dielectric constant due to polarization (electrostatic) effects across the interface [37, 38].
When the above methods are compared, different results are found for an identical sample. The charge mobility determined by the TOF method is often found to be lower than the PR-TRMC value. This is because TOF mobility is measured over relatively thick samples (usually tens of microns) on a millisecond or microsecond timescale and therefore is more susceptible to defects and grain boundaries. Nevertheless, TOF experiments lead to higher mobility values over OFET and SCLC techniques, which yield one order of magnitude lower. This discrepancy is because the carrier injection efficiency is lower between the metal electrodes and the organic material in both SCLC and OFET devices [39].
Furthermore, charge transport behavior in DLC materials with multiscale conformational dynamics were computationally investigated [40]. From that, fundamental understanding of the relationship between the mechanism of charge transport and structures of the active organic materials, including chemical composition and supramolecular organization, has been well established, which is critical for improving device performance [41]. By improving molecular organization, the mobility of charge carriers along molecular stacks can be significantly enhanced. Therefore, to facilitate efficient charge transport, developing ordered self-assembling materials with defined structures is highly desired.
2.3 Discotic Molecular Systems
For the DLCs, the structure is the most significant and direct factor influencing the supramolecular assembly, which leads to multiple morphologies, properties, and functionalities. To develop novel functional DLC materials and/or to improve the performance of devices using these materials, understanding the structural effects and structure–property relationships is fundamental. To improve the semiconducting properties of DLCs, creating versatile DLC materials is a key issue. A typical DLC molecule is a model having a rigid aromatic core surrounded by multiple flexible side chains. With organic synthesis, the main driving force to the structural diversity, it is feasible for researchers to vary the nature of the central core and the peripheral substituents. Increasing the DLC core size, which enhances the π-orbital overlap within the columnar structure, is one of the most successful designing strategies that have been widely accepted to achieve high charge-carrier mobility. To further strengthen the π–π stacking of molecules, various ways have been explored to enhance the inter-disc correlation within the columns, for example, introducing hydrogen bonding and dipole–dipole interactions. On the other hand, flexible chains on the periphery of discotic molecules help introduce liquid crystalline properties and processability (solubility). When the chain length and structure change (e.g., with branches or/and heteroatoms), the resulting phase behavior and alignment will be altered. On all accounts, by varying molecular structures, DLC materials with good thermal and chemical stability as well as desirable electronic properties (appropriate HOMO and LUMO energy levels) can be obtained. In this section, the molecular structures of common DLC materials will be presented.
2.3.1 General DLC Molecules with High Charge Mobility
Most discotic molecules have rigid large-aromatic cores. The typical core structures varying from small size to large size are listed in Figure 2.7 in the order: benzene [2, 42], triphenylene [43], pyrene [44], perylene [12], porphyrin [45], phthalocyanine [46], and hexabenzocoronene [47].
Figure 2.7 Typical aromatic structures for discotic cores.
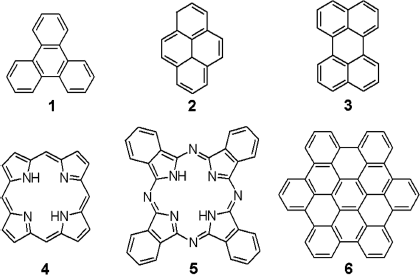
Benzene is the smallest and the first used aromatic core for discotic mesogen [48]. Since researchers have discovered that larger aromatic cores tend to form more efficient π-stacks, extending the sizes of the conjugated cores has become one important way to develop new discotic mesogens. In 1982 Simon and co-workers first discussed the idea of using discotic liquid crystals as quasi-one-dimensional conductors [49]. In the early 1990s, researchers successfully generated photo-induced charge carriers in chemically pure DLC molecules and measured the mobility by PR-TRMC or TOF technique. In 1993, Adam et al. managed to measure photo-induced charge-carrier mobility of 1 × 10−3 cm2 V−1 s−1 in the columnar phase of hexa-pentyloxytriphenylene 7 by TOF technique (Figure 2.8) [22]. Further in 1994, they discovered one DLC material hexahexylthiotriphenylene showing a decent charge carrier mobility (holes μ+) of ca. 0.1 cm2 V−1 s−1 [50]. From these studies, valuable information of charge transport in DLCs was accomplished for the first time, via examining the nature of charge carriers and observing charge transport along the column axis. After that, the major focus of research on DLCs has proceeded from the beginning studies of structure–property relationship to the advanced improvement of charge transport, in order for developing new systems for various practical applications with higher mobilities.
Figure 2.8 Molecular structures of disc-like triphenylene derivatives.
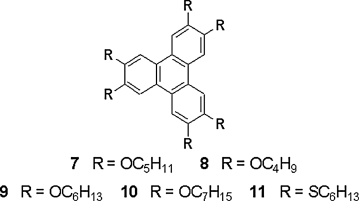
Triphenylene derivatives as shown in Figure 2.8 are typical DLC materials. Their properties are significantly influenced by the nature of the substituents. For example, a strong tuning effect in the order within the columnar phase of discotic triphenylenes is achieved by varying only one of the lateral chains on the aromatic core, resulting in dramatic changes of phase behavior and photophysical properties [51, 52]. A systematic charge mobility study of triphenylene-based DLC molecules indicated that the charge transport properties are strongly correlated with the order in the mesophases. For DLC 7 in the Colh phase, carrier mobilities (holes) of the order of 10−3 cm2 V−1 s−1 were measured [22]. For a smaller homolog 8, approximately an order of magnitude increase in the hole transport was observed suggesting the existence of more ordered plastic columnar phase [53]. Compared with the analog compounds 9, 10 with longer-side chains, the shorter-side chain derivatives 7, 8 have better π-orbital interactions of the cores, resulting in higher mobilities [54]. Interestingly, even a higher hole mobility value (1 × 10−1 cm2 V−1 s−1) has been found in DLC 11 with higher mesophase order when the alkyl chains connect to the core via sulfur. These DLC molecules provide generally higher charge mobility values than those with the chains linking either directly or via oxygen to the cores [50].
In addition, compound 11 has been used to show the correlation between the carrier mobility and molecular order of DLC materials, as displayed in Figure 2.9. During cooling, 11 exhibits phase transitions from isotropic to Colh phase, to the more ordered columnar helical phase, and eventually to the crystal phase below 40°C. In the isotropic liquid phase, a low charge mobility value of 1 × 10−4 cm2 V−1 s−1 is measured because there is no columnar order. When order increases, the value rises to 5 × 10−3 cm2 V−1 s−1 in the Colh phase and further to 0.1 cm2 V−1 s−1 in the columnar helical phase. Furthermore, although in the crystalline phase charge-carrier mobility could not be determined by the TOF technique due to the trapping sites at grain boundaries, the sum of the one-dimensional charge-carrier mobilities (about 0.4 cm2 V−1 s−1) has been derived from the PR-TRMC technique [23]. By combining the results of both techniques (TOF and PR-TRMC), an elevation in mobility by more than three orders of magnitude has been achieved during transitions from mesophase to crystalline phase. This result is a common feature of a number of different discotic materials and is attributed to the more ordered packing of molecules.
Figure 2.9 The charge-carrier mobility in different phases of 11 measured by TOF (μh; squares) and sum of one-dimensional intracolumnar mobility Σμ1D (open circles) estimated by PR-TRMC experiments while cooling the sample from its isotropic phase. Cr: crystalline phase; H: helical columnar phase; Colh: hexagonal columnar phase; I: isotropic phase.
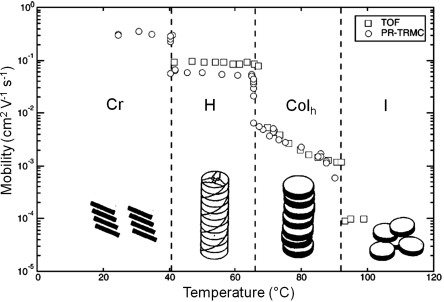
For organic semiconductors, a vast majority of the known discotic mesogens are relatively rich in electrons, which are better for transporting holes (p-type) than electrons (n-type). For discotic triphenylene derivatives, earlier studies have reported that the majority of them were p-type semiconductors and the electron mobility was much smaller than the hole mobility, indicating the major charge carriers are holes. However, recent research has discovered that by improving the purity of materials, ambipolar charge transport (for both electron and hole) in the columnar phases of 7 and 11 could be obtained [55, 56]. This is important because electron-transporting materials (electron acceptors) with high mobility are essential for solar cells, bipolar transistors and organic light-emitting diodes (OLEDs). For example, a layer of n-type semiconducting material sandwiched between the cathode and the emission layer enhances the performance of LEDs by facilitating the electron injection. More efforts have been devoted to synthesizing and characterizing electron-deficient (n-type) discotic molecules recently as n-type materials are highly desired but are fewer compared to the large number of electron-rich (p-type) ones. From the molecular structural point of view, connecting electron-withdrawing peripheral groups onto a p-type discotic core or modifying aromatic core structures to be electron-deficient (e.g., with heteroatoms) are feasible ways. 12 is one example of n-type DLC materials built up with hexaazatriphenylene as core structure connected by electron- withdrawing carbonyl substituents (Figure 2.10) [98]. Meanwhile, substitution of electron-withdrawing groups can lower the LUMO and HOMO levels in π-conjugated systems, which reinforces electron mobility.
Figure 2.10 Molecular structures of triphenylene derivatives with various modifications 12–16.

Lehmann et al. reported that DLC hexaazatrinaphthylene (HATNA) 13 with an electron-deficient core showed charge carrier mobilities of 0.3 cm2 V−1 s−1 by PR-TRMC measurements and demonstrated the electron-deficient character for 13 by cyclic voltammetry [57]. Hexaazatriphenylene 14 is another DLC example with an electron-deficient aromatic heterocyclic core. When it was blended in films with a donor material poly(3-hexylthiophene) (P3HT), the photo-induced electron transfer process has been investigated and Meijer et al. determined it as an electron-acceptor [58]. As a matter of fact, electron transport in the columnar mesophase was first evidenced in an electron-deficient thioether substituted tricycloquinoxaline derivative 15 (Figure 2.10) [59], which was similar to triphenylene core in shape. Conductivity of about 2.9 × 10−5 S m−1 in the columnar phase was obtained and later when replacing the hexylthio side chains with ethyleneoxy chains (16), the conductivity reached to an even higher value of 1.1 × 10−3 S m−1.
Among the n-type DLC materials, one class showing particularly high charge mobilities are the perylene derivatives shown in Figure 2.11, which are often functionalized with substituents at the imide N atoms. Perylene diimide (PDI) compounds have strong optical absorption and excellent thermal, chemical, and photochemical stability. They are widely used as pigments and dyes in industry. Recently, discotic mesogens of PDIs rose as an attractive n-type DLC semiconductor. Due to their photoconductivity and n-type semiconducting properties, these materials have significant applications in prototype devices such as solar cells, OLEDs, and OFETs. In recent reviews by Würthner [60] and Langhals [61], details have been discussed in synthesis, supramolecular self-assembly and spectral properties of PDI derivatives and related compounds. For PDI derivatives with relatively short N-substituents, they are crystalline solids with high melting points. When substituents at the two imide nitrogen atoms increase to a sufficient length, liquid crystalline phases (e.g., 17–20) may appear. When operated under nitrogen atmosphere, 18 in thin-film transistors showed a field-effect mobility as high as 0.6 cm2 V−1 s−1 [62]. As the first reported perylene-based DLC compound for charge transport mobility by PR-TRMC method, 21 illustrated mobilities of 0.1 cm2 V−1 s−1 and 0.2 cm2 V−1 s−1 in the liquid crystalline and crystalline phases, respectively [63]. When the substituted chains further become branched, for example, compound 22, which bears trialkoxyaryl substituents at N atoms, the LC mesophase can enter room temperature with a wide transition range from room temperature to 373°C [64]. When an additional CH2 spacer was introduced between the tri(alkoxy)phenyl group and the imide nitrogen atom in the molecule 23, the clearing point was reduced to 226°C, making it easy to process from the melt. Under ambient conditions, it is intriguing to find that this compound shows an electron mobility of 1.3 cm2 V−1 s−1, even higher than that of amorphous silicon [12]. In order to further induce mesomorphism, modification of PDI cores at bay positions was considered by researchers. For example, when a bulky substituent was introduced to the bay area (scaffold) of the molecule 24, the isotropization temperature further decreased to 283°C. Also, charge transport properties were enhanced for tetrachloro-substituted perylene bisimide 25, comparing to a non-substituted analog compound at bay positions [65]. DLC PDIs were used in OLEDs because of their strong emission in columnar mesophases. Red light emission by combining electron-deficient 26 with electron-rich triphenylene 17 in a bilayer OLED device was demonstrated by Destruel et al., since fluorescence in the columnar mesophase of perylene tetraester compound 26 was discovered [66]. Another example of an OLED was reported by combining columnar LC 27 having strong electron-withdrawing substituents with benzoperylene triesters, which yielded a red color emission [67].
Figure 2.11 Molecular structures of perylene derivatives 17–30.
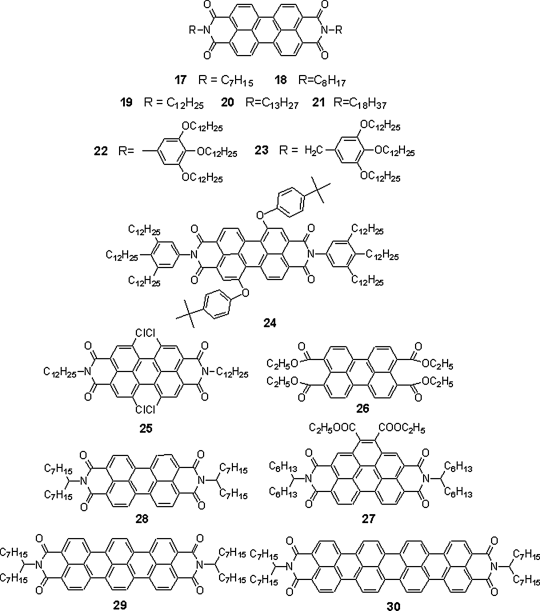
Since larger aromatic cores may bring highly ordered supramolecular organizations which enhance charge carrier mobilities, extended PDI aromatic cores along the long molecular axis have led to the investigations of terrylene diimides (TDI) and quaterrylene diimides (QDI). With identical branched substituents connected to the cores, 28, 29 and 30 were synthesized [68]. When core size enlarges from 28 to 30, the isotropization temperature increases from 130°C to >500°C. Although 28 and 29 directly melt from a crystalline state to the isotropic liquid without forming any LC mesophase, 30 with the largest aromatic core is able to form hexagonal columnar phases above 188°C.
Porphyrin has a larger aromatic core and the macrocycle has 26 π electrons. The heterocyclic macrocycles are composed of four modified pyrrole subunits connecting at their α carbon atoms via methine units. Porphyrins are important natural materials: two well-known porphyrins are chlorophyll, an important component in green leaves, and heme, the pigment in red blood cells. For applications, incorporating porphyrin derivatives into photovoltaic devices is highly attractive because of their unique light harvesting ability which has been proved by efficient photosynthesis in green leaves, which evolves by hundreds of million years' natural selection. Moreover, porphyrins are becoming more and more important in science and technology owing to their thermal stability, excellent charge transport ability due to large π-stacks, and photochemical properties. Phthalocyanine has a similar core structure to porphyrin but with four phenyl group combining to pyrrole units and the carbon bridge units changing to nitrogen atoms. When π-electron-rich macrocycles of porphyrins and phthalocyanines are substituted at the periphery by alkyl chains, they tend to self-assemble and form columnar mesophases which have received much attention [49, 69–74]. In fact, porphyrin and phthalocyanine derivatives were the first DLCs samples investigated for the charge transport by PR-TRMC method [75, 76]. As studies have revealed that the substituting chain structures and metal ions have influences on phase transition temperatures, mesophase ranges, and carrier mobilities, the liquid crystalline properties and charge transport ability can be tuned by desirable molecular structures via versatile synthesis.
Recent research on DLC porphyrin derivatives has provided some important supplements to self-organized charge transport materials for photovoltaic devices. Our group has designed and synthesized some porphyrin-based DLCs (e.g., 31–35 as shown in Figure 2.12) which combine easy synthesis, high chemical purity, and unique self-aligning properties, together with a wide DLC temperature range for their mesophase, extending even to room temperature [77]. It is intriguing to point out that they are able to spontaneously form defect-free large-area monodomain films with homeotropic alignment. This is beneficial to potential photovoltaic applications [78]. Moreover, we have achieved solution processable bilayer- and bulk-heterojunction solar cells based on 31–32 and the resulting power conversion efficiency achieved under ambient conditions appears to be higher than any other reported solar cells using columnar DLC materials [79].
Figure 2.12 Molecular structures of DLC porphyrin derivatives 31–35.
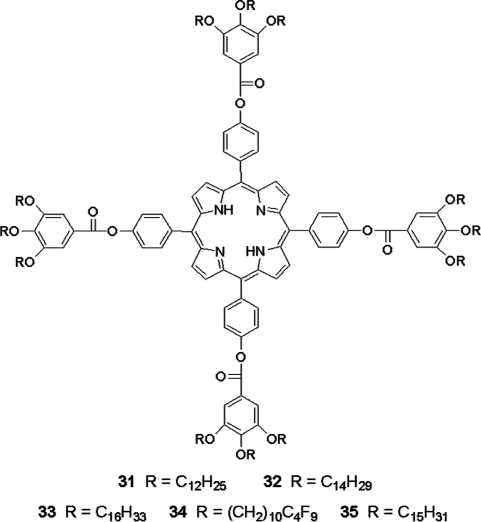
For phthalocyanines, early charge-carrier mobility measurements of 36, 37 (in Figure 2.13) have been carried by PR-TRMC, revealing charge mobility values over 0.1 cm2 V−1 s−1 [75]. For 37 when it is combined with electron-deficient peripheral alkylsulfonyl substituents, it exhibited a first reduction potential value of about −0.14 V versus the saturated calomel electrode (SCE), which indicated this material as a potential air-stable n-type semiconductor [80]. Bushby et al. recently reported high hole mobility (~0.2 cm2 V−1 s−1) in the Colr phase of an octaalkyl phthalocyanine 38 [81].
Figure 2.13 Molecular structures of phthalocyanines 36–38.
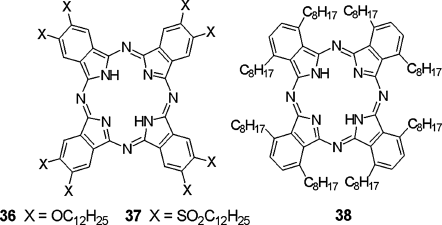
When the aromatic core is further extended, a planar aromatic molecule HBC 6 forms, consisting of 13 fused six-membered aromatic rings. HBCs and their larger derivatives usually possess sixfold (D6h) symmetry and stack with an average rotational degree of 30° in their columnar phases. K. Müllen's group has extensively investigated this class of materials [82]. HBCs are attractive materials for semiconducting applications such as OFETs and solar cells [83], because they have planar aromatic discogens with large π orbitals, forming highly ordered columnar mesostructures not only presenting a large mesophase range that extends to room temperature [84], but also providing outstanding charge carrier mobility values reaching 0.38 cm2 V−1 s−1 [26]. For the corresponding crystalline phase, charge mobility values can exceed 1.1 cm2 V−1 s−1 [28].
HBC derivatives have also been developed. Since a theoretical prediction has pointed out that molecules of threefold symmetry (e.g., triangular shaped polycyclic aromatic hydrocarbons (PAHs)) with a helical packing structure and 60° as rotation angle would form optimal local arrangement for higher charge mobilities [85], DLC triangle-shaped 39, 40 in Figure 2.14 were synthesized, reporting improved photovoltaic performance in comparison to the other HBCs [86].
Figure 2.14 Molecular structures of modified HBCs 39–42.
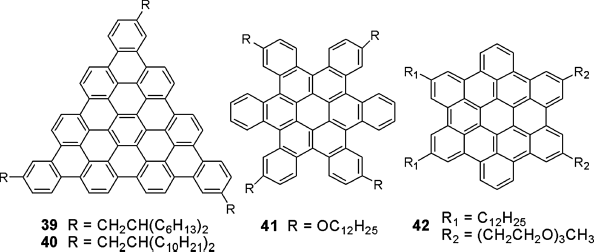
Furthermore, C. Nuckolls et al. reported a contorted HBC derivative 41 with stable columnar mesophase [87]. It formed LC films in OFETs, exhibiting relatively high carrier mobilities and current modulation (μ = 0.02 cm2 V−1 s−1; on–off current ratio of 106:1). A gemini-shaped HBC derivative 42 was created by Aida et al. This structure has two hydrophobic dodecyl chains on one side and two hydrophilic triethylene glycol chains on the other [88]. Its solution cast films exhibited a hole mobility of about 1.0 × 10−4 cm2 V−1 s−1.
For further improving the charge transport properties of these materials, the HBC derivatives with even larger PAHs was synthesized, and some of the resulting DLC materials provided high charge mobilities [89]. In Figure 2.15, the PAH rings contain a larger number of aromatic carbons varying from 24 to 132 [89–92]. They are like graphite structures. With these compounds, a relationship between the sizes of the conjugated aromatic cores and the corresponding charge transport abilities has also been discussed [83].
Figure 2.15 Chemical structures of disc-like PAHs of various core diameters: 43 (ca. 1.0 nm), 44 (ca. 1.6 nm), 45 (ca. 1.9 nm), 46 (ca. 2.0 nm), and 47 (ca. 2.3 nm).
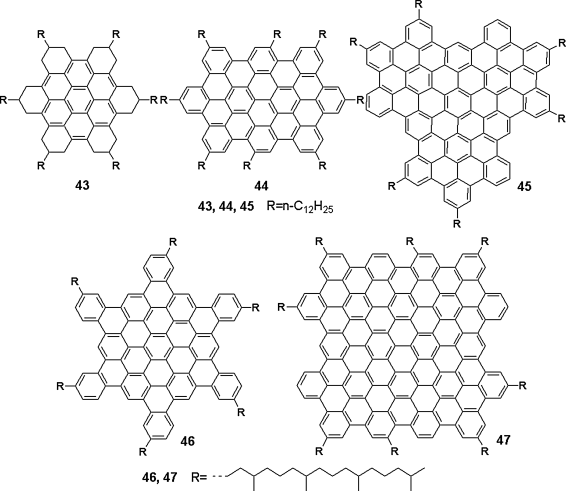
Apart from the satisfying charge transport properties, DLC PAH materials have also exhibited excellent optical properties which are important for applications in photovoltaic devices, because absorbing sunlight efficiently over a large range of wavelengths, in other words, matching the sun flux spectrum is highly desired. This target can be achieved by extending the aromatic core of PAH as the maximum absorption peak shifts prominently when the size of aromatic cores increases. There is an empirical formula from experimental results: λmax = 280 + 2n, where n is the number of carbon atoms in the aromatic core. After the absorption peaks red shift, together with the broadened peaks, a wider coverage to sunlight could be achieved [83].
On the other hand, although large PAH structures show advantages for various applications, there are problems of purifying these molecules with very big aromatic cores. The traces of impurities are charge trapping sites which destroy free charges and the mobility values could be undervalued from experimental results. Additionally, not all of these graphites like PAH rings are planar. In recent years, with further modification to the structures of HBC cores, some of the novel multi-aromatic compounds are ring bent and became non-planar [93].
Apart from the above commonly known DLC materials, a few other cores like coronene, decacyclene, and rufigallol are also used. With appropriate substituents, the corresponding materials exhibit charge transport in their DLC mesophase [94–96].
2.3.2 H-Bond Assisted DLCs
Introducing intermolecular hydrogen bond may be an efficient way to enhance mesophase order and charge transport in DLC materials. It can anchor the molecules in DLC columns. For example, peripheral functionalized triphenylene derivative C18H6(OC6H13)4(OCH2CONHR)2 containing amide groups at the 2,7-positions, which have H-bond interactions with adjacent molecules in columnar stacks, has been synthesized. As shown in Figure 2.16, the intermolecular hydrogen bonds stabilize the columnar organization and lead to an ordered mesomorphic and organic gel state [97]. For other examples, Gearba et al. reported a short interdisc distance of 3.18 Å for 12 which is the smallest value ever found in the columnar phase, due to the presence of intermolecular hydrogen bonding. As a result, an enhanced Σμ1D mobility up to 2.0 × 10−2 cm2 V−1 s−1 in the columnar mesophase of hydrogen bonded hexaazatriphenylene 12 was found [98]. Due to the presence of intracolumnar hydrogen bonding, an interesting discovery of benzotristhiophene derivative 48 having a temperature independent charge mobility (2.0 × 10−2 cm2 V−1 s−1) in two hydrogen bonded thermotropic hexagonal columnar mesophases was reported (Figure 2.17), even across phase transition between the two mesophases [99].
Figure 2.16 Hydrogen bonds anchor the columnar organization resulting in more ordered Colh mesophase and organic gel.
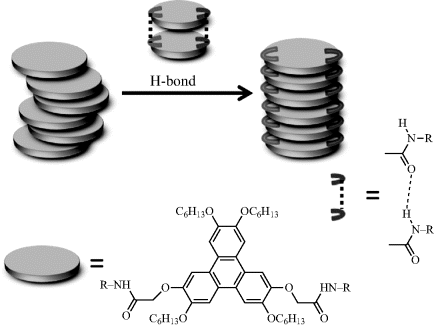
Attributed to the highly ordered columnar hexagonal plastic phase that was induced by the intermolecular hydrogen bonding of the 1,3,5-benzenetrisamide with three pendant hexaalkoxytriphenylene groups, a high charge mobility of 0.2 cm2 V−1 s−1 was reported [100]. Reported by Kato et al., even by mixing with hydrogen-bonded fibers forming physical gelation of molecules, molecular fluctuations in the columnar phase was suppressed successfully and a threefold enhancement in the hole mobility of 9 was obtained [101].
Figure 2.17 Chemical structures of 48–50, and schematic columnar stacks of 50.
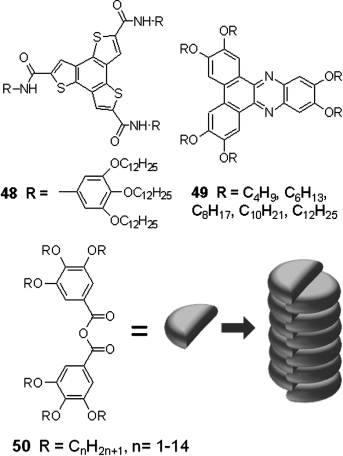
Similarly, dipole–dipole interactions can also be applied to the design of self-assembled discogens. For example, hexaalkoxydibenzo[a,c]phenazines (HDBP) 49 possess stable hexagonal mesophases in a fairly broad temperature range and can form homeotropically aligned films [102]. 3,4,5-Trialkoxybenzoic anhydride 50 forms a columnar mesophase with geared interdigitation through polar junctions among molecules (Figure 2.17) [103].
Furthermore, by using hydrogen-bonding interactions, supramolecular self-assembled discogens forming disc units in a columnar phase have been obtained, for example 51 in Figure 2.18. This provides a way to create larger molecular discogens for potentially improving charge carrier mobilities. Kato et al. have recently reviewed many other examples of mesogenic H-bonded systems, and useful applications of the resultant supramolecular structures have been highlighted [104, 105].
Figure 2.18 Cyclic disk-like aggregation structure 51 composed of four hydrogen-bonded self-assembled folic acid derivatives (pterin molecules).
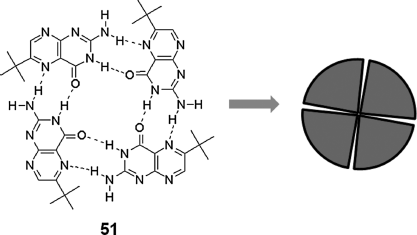
2.3.3 DLCs with Partially Perfluorinated Periphery
In recently reported research, there is an interesting concept in the design of discotic semiconductors using peripheral polyfluorinated alkyl chains, although there is enormous previous research in fluorinated calamitic liquid crystals [106]. For DLC materials, fluorinated substituents are expected to create an inert “mantle” around the discotic core that effectively increases the columnar phase stability. As the melting point becomes higher, one dimensional charge transport is improved because of the reduced intercolumnar interactions. For polyfluoroalkyl triphenylenes 52, it is shown that introduction of polyfluoroalkyl groups favors the formation of spontaneous homeotropic alignment (Figure 2.19) [107]. Also, other DLC molecules with partially perfluorinated alkyls such as porphyrin 34 [77(b)] and HBC 53 [108] have been obtained. They are expected to not only become more stable in DLC phase, but also show better alignment and reduce lateral conduction, and hence improve one-dimensional charge carrier mobilities.
Figure 2.19 Top: Chemical structures of 52 and 53. Bottom: Schematic drawing of homeotropically aligned columnar structures of fluorinated triphenylene 52.

2.3.4 Incorporating Fullerenes into DLCs
It is known that fullerene and its analogs are one of the most important families of organic semiconductors. In the past years, numerous electron donor–acceptor complexes with fullerene as the electron acceptor core have been synthesized to demonstrate the electron transfer process. In organic photovoltaics (OPVs), the formation of a bicontinuous interpenetrating network from donor and acceptor components as a result of self-organization on the nanometer scale is one key issue for efficient carrier transport of the separated charges. The use of fullerene combined with liquid crystals to form a self-organized phase can be an important approach to improve device performance [109].
Of all the donor–acceptor blends, the porphyrin–fullerene blend is an attractive combination since fullerene is an excellent electron acceptor, and porphyrin is a superior electron donor. Recently, it was found that the blend of porphyrin and PC61BM (a fullerene derivative) can self-organize into a highly ordered thin film by thermal annealing as shown in Figure 2.20 [110]. The hexagonal columnar phase of porphyrin with PC61BM at interstices of the columns has been proposed as the molecular arrangement or it is possible that PC61BM is sandwiched between two porphyrin cores because of the strong π-donor and π-acceptor interaction. Regardless of the packing arrangement, a homeotropically aligned columnar architecture of the porphyrin and PC61BM complex has been proved by XRD. This is very important for OPV applications because such a molecular arrangement can provide an efficient path for electrons and/or holes along the columnar axis where the light harvesting molecules are arranged with the largest area toward the incident light.
Figure 2.20 Top: Synchrotron XRD patterns from homeotropic monodomain of material 35 (a) and the blend of 35 (b) with PC61BM in an 8-µm-thick glass cell. Bottom: Calculated geometric dimensions of porphyrin 35 and 3D ChemDraw spacing-filling model of fullerene derivative PC61BM and the schematic representations of homeotropically aligned architecture of the blend of 35 and PC61BM. Reproduced with permission from ref. 110. (See the color version of this figure in Color Plates section.)
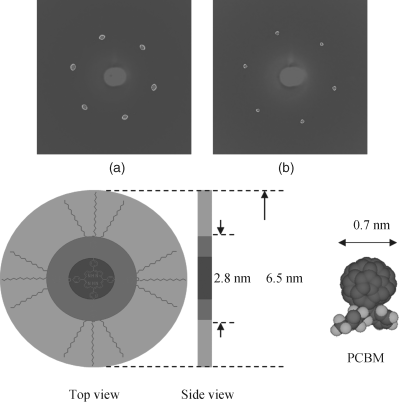
Besides, the C60 unit can be connected to electron donor molecules by covalent bonding. One example of phthalocyanine–C60 dyad 54 (a mixture of isomers, details see Ref. 111) by connecting PC61BM and phthalocyanine derivative designed for heterojunction OPV cells was reported by Geerts et al. (Figure 2.21) [111]. The dyad exhibited a LC phase at room temperature.
Figure 2.21 Chemical structure of phthalocyanine–C60 dyad 54.
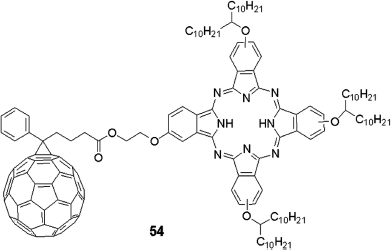
Moreover, intermolecular interaction is another way that links fullerene to electron donor molecule. Guldi et al. observed a very strong electronic communication through hydrogen bonds between porphyrin and C60 forming 55 in Figure 2.22 [112], which exhibited a longer-lived formation of radical-ion-pair state (~10 µs in THF) compared to that of the similar covalent C60 conjugates (~1 µs in THF) [113]. It showed an even higher lifetime of 2.02 µs [114] for the photogenerated radical-ion pair due to the stronger Watson–Crick hydrogen bonding in 56 [115–118]. Later, phthalocyanine has also been incorporated into this kind of structure [119].
Figure 2.22 Chemical structure of the fullerene-based hydrogen-bonded donor–acceptor ensembles 55 and 56.
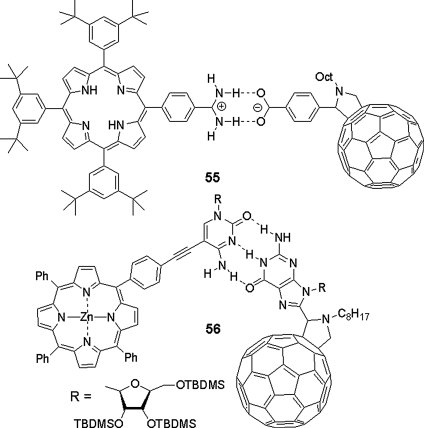
2.3.5 Incorporating Metal Elements into DLCs
Metal elements can be combined together with organic materials through metal–electron pair coordination and they have an important impact on organic semiconducting materials. On one hand, liquid crystal phases can be induced by the presence of a metal complex. The effect of a metal enhances the mesophase behavior of the material. In the area of photovoltaic cells, researchers clearly want to mimic chlorophyll to have its light-harvesting and electron-transfer properties. The liquid crystal property of this kind of metal–porphyrin complex was first reported by Bruce's group [120]. On the other hand, the metal ion can also change the electronic property of the material and affect the charge transport along the column. For example, metalations of the porphyrins will change the molecular energy and alter the absorption as well as photoemission spectrum. Many metallo-porphyrins or phthalocyanines coordinated with transition metal complexes have been reported, presenting attractive photovoltaic effect [75, 121]. Ohta et al. found ambipolar charge transport (a negative charge mobility of 2.4 × 10−3 cm2 V−1 s−1 and a positive mobility of 2.2 × 10−3 cm2 V−1 s−1) in the Colh of a Cu-phthalocyanine compound 57 [122]. Cook et al. recently reported a high hole mobility of 0.2 cm2 V−1 s−1 in the Colr phase of an octaalkyl phthalocyanine 38 [123]. Higher mobility values as high as 0.7 cm2 V−1 s−1 were reported in the columnar phase of phthalocyanine metallomesogens [124].
Aida et al. recently synthesized a fused metalloporphyrin dimer 58 (Figure 2.23) bearing hydrophobic (alkyl) and hydrophilic (triethylene glycol) chains in the periphery and reported its self-organization into a room-temperature columnar phase. High electron mobilities were found for the LC film of 58 (0.27 cm2 V−1 s−1 at 16°C) [125].
Figure 2.23 Molecular structure of porphyrin derivative 57 and dimer 58.
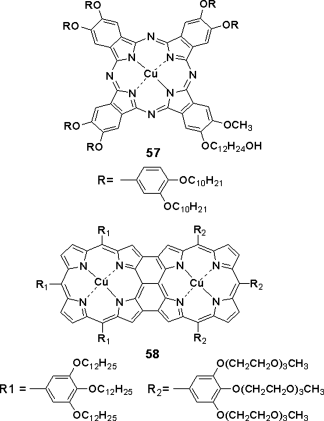
Apart from common transition metals, porphyrin complexes with lanthanide ions (Eu3+, Tb3+, Dy3+, and Sm3+) [126, 127] have been studied (Figure 2.24). They exhibit unique optical properties. For example, they exhibit line-like emission bands and relatively long luminescence lifetimes [128–131]. Recently, holmium(III) Ho3+ porphyrin complexes 59 have been developed, which present the Colh phase and have an extended lifetime of excited state [132].
Figure 2.24 Molecular structure of holmium porphyrin complexes 59 and phthalocyanine complex 60.
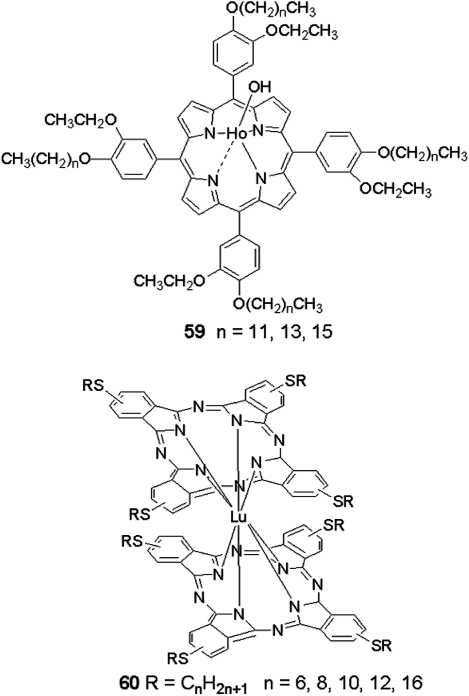
Not only porphyrin but also other mesogens can coordinate with lanthanide ions forming DLC structures, showing intriguing properties, for example, phthalocyanine 60 (Figure 2.24) [133] and N-aryl based Schiff bases (Figure 2.25) [134].
Figure 2.25 Molecular model of the ligand and complex in disc-like form, which can organize in columnar phase. Reproduced with permission from ref. 134.
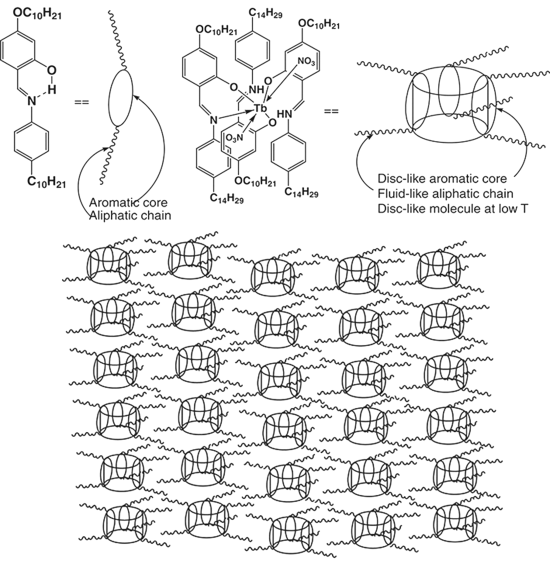
2.3.6 Incorporating Gold Nanoparticles (GNPs) in DLCs
Very recent studies revealed that doping gold nanoparticles into the columnar phase does not disturb the nature of the columnar mesophase (only alter the transition temperatures), but improves charge transport behavior significantly. For the DLC material 2,3,6,7,10,11-hexakis-hexyloxy-triphenylene, the presence of 1% (w/w) of methylbenzene thiol coated gold nanoparticles increases the conductivity of the discotic liquid crystal by about two orders of magnitude [135].
For more research on incorporating gold nanoparticles into the supramolecular order of DLCs, Kumar's group has synthesized GNP stabilized by simple alkanethiols, and dispersed them into the columnar matrix of different DLCs. Thermophysical properties of these nanocomposites have been studied and the columnar phases of DLCs could well-disperse the nanoparticles in their matrices. However, discotic nematic liquid crystal did not disperse well the nanoparticles and there was macroscopic phase separation [136, 137]. Remarkably, there is a dramatic increase in DC conductivity of DLC hexaheptyloxytriphenylene (H7TP) by more than 106 times upon doping with 1% triphenylene-GNP (TP-GNP) [138]. The GNP doped molecular stacking columnar structure is shown in Figure 2.26. Similarly, carbon nanotubes have also been mixed into DLC mesophases. As a result, LC nanocomposites with broad mesophase ranges and different electronic properties may potentially be important for many device applications, such as photoconductors, light-emitting diodes, photovoltaic solar cells, sensors, thin-film transistors [139].
Figure 2.26 A schematic diagram illustrating TP-GNP structure and its arrangement in the inter domain spacing formed between the discotic columns. Reproduced with permission from ref. 138.
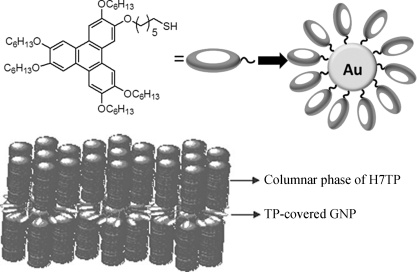
2.4 Alignment of DLC Materials in Active Semiconducting Layers
For DLC materials, one key issue determining charge-carrier mobility is the order of the molecules in columns. Therefore, efforts have been devoted to discover processes leading to highly ordered monodomain thin-films of DLC materials. The charge transport mechanism in columnar phase is one fundamental point to know, which indicates DLCs are quasi one-dimensional semiconductors and charges travel much faster along the columnar stacks than between columns. This implies that molecules must be appropriately aligned in a column to form a tunnel that the current can go through. According to the aligning direction of DLC molecules on a substrate surface, homeotropic alignment (or “face-on” orientation) means columns are perpendicular to the surface and planar alignment (or “edge-on” orientation) means columns are parallel to the surface have been defined. The models are shown in Figure 2.27. The first alignment is preferred by solar cells, OLED devices and the second is for OFET devices. In such devices, the conducting columns are usually generated as defect-free long-range ordered thin (a few tens of nanometers) films to fill the gap between the anode and the cathode. Although numerous discotic molecules that can self-organize into columnar structures have been synthesized, controllable molecular orientation either forming homeotropic or planar alignment onto a substrate is difficult. Conventional techniques used for alignment of calamitic LC phases are not useful for the highly viscous columnar LC materials [140]. Therefore, new device fabrication techniques are in strong demand for organizing discotic molecules, forming homeotropic or planar alignment.
Figure 2.27 Schematic representation of the homeotropic (left) and planar (right) orientations of a discotic columnar mesophase.
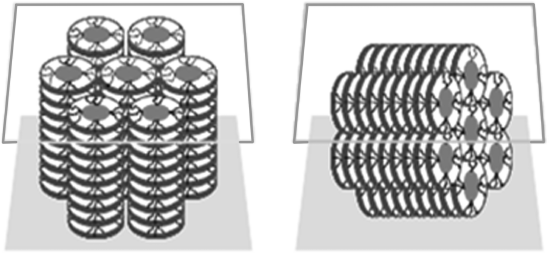
2.4.1 Homeotropic Alignment
A “face-on” alignment of the columnar mesophase sandwiched between two substrates can generally be generated by thermal annealing. Various examples such as triphenylene, phthalocyanine, porphyrin, and hexabenzocoronene DLCs have exhibited such an alignment in their columnar mesophases in relatively thick films (even a few micrometers) when the material is confined between two substrates and slowly cooled from their isotropic state. For example, a systematic study of one DLC triphenylene derivative on various substrates revealed that the homeotropic alignment is thermodynamically favored in the columnar phases [141]. It is noteworthy that a slower cooling rate is more favored because the high viscosity of the columnar mesophase often quickly promotes multidomains and prevents the perfect homeotropic alignment over a large area. In recent years, the development of homeotropically aligned DLC materials has become more comprehensive for the influences of different parameters such as molecular structures and surface interactions. For example, by incorporating heteroatoms in the flexible side chains or by introducing groups with larger sterical hindrance on to the mesogenic core, the isotropic melt viscosity of mesogens has decreased. Thus, developing novel ways for various DLCs to obtain homeotropic alignment has been one attractive research topic. Researchers have used mixed molecules forming self-organized DLCs for high-efficiency organic photovoltaics [142]. A face-on oriented bilayer heterojunction formed by two types of discotic molecules was reported (Figure 2.28) [143]. These designed molecules have specific properties, e.g., selective solubility, low degree of miscibility, controllable transition temperatures, and room temperature hexagonal columnar liquid crystallinity.
Figure 2.28 (a) Schematic structure of the two compounds 1′ (where only one of the two regioisomers is sketched) and 2′. Their low degree of miscibility is shown by a contact preparation between coverslip and glass slide in the isotropic liquid phase. Observation performed by bright field microscopy. The image size is 440 µm × 325 µm. (b) Growth by thermal annealing of a homeotropically oriented open bilayer (cooling rate: 5°C/min) observed by differential interference contrast microscopy. In a preliminary step, both compounds 1′ and 2′ have been sequentially deposited by spin-coating before (a) being heated up to their isotropic liquid phase (Iso1′ and Iso2′, respectively). (b and c) Nucleation of the compound 1′ in the columnar liquid-crystalline phase (CLC1′) with a dendritic texture, while the upper layer (compound 2′) is still in its isotropic liquid phase (Iso2′). (d and e) Growth of CLC2′ above CLC1′. Note the apex angle of the CLC2′ growing domain of ≈120° characteristic of a face-on alignment. (f) Both layers exhibit a homeotropic alignment, as confirmed in the inset by the lack of birefringence between crossed polarizers. The layer thicknesses are approximately 350 and 200 nm for compounds 1′ and 2′, respectively. The image size is 715 µm × 650 µm. Reproduced with permission from ref. 143.
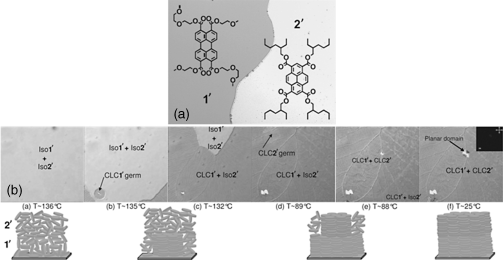
An effective method to obtain homeotropic alignment is to modify the affinity of molecules by changing the chemical nature of the side chains to the substrate surface such as polyimide, ITO, and cetyltrimethylammonium bromide (CTAB)-coated glass substrates. Partially perfluoroalkyl groups were used as the peripheral chains for triphenylene mesogens, promoting homeotropic alignment in the hexagonal columnar phase [144]. Porphyrin derivative 34 with partially perfuoronated alkyl chains on peripheral [77(b)] can form homeotropic alignment on various substrates as well, which is more important as it has a larger π-conjugated system, together with other advantages of porphyrin (Figure 2.29). On the other hand, there are reports demonstrating homeotropic alignment of columnar mesophase on surfaces with certain nature or treatment. For example, Pisula et al. reported spontaneous homeotropic alignment of branched long alkyl chain-substituted HBC derivatives on ITO substrates [145]. There were reports on the formation of homeotropically aligned open films of a hexagonal columnar phase on discotic phthalocyanine derivatives deposited on ITO substrates treated by either UV, ozone or nitrogen plasma [146, 147]. Geerts et al. also demonstrated a method of using a polymer sacrificial layer to induce homeotropic alignment of the columnar LC films, as shown in Figure 2.30. In their method, homeotropic alignment of phthalocyanine is imposed by thermally annealing the confined LC film with a top polymer layer that is later removed by washing with a selective solvent [148]. Moreover, ordered porous alumina templates have been introduced to prepare homeotropically aligned nanowires of a triphenylene discotic compound, reported by Steinhart et al. [149]. One interesting observation was about introducing homeotropic alignment of corannulene-based DLC molecular columns by applying an electric filed, considering that most known DLCs so far were not responsive to the applied electric field in their columnar mesophases [150]. Furthermore, alignment change of the discotic liquid crystal domains induced by infrared irradiation was recently investigated for the hexagonal columnar phase of hexahexylthiotriphenylene, as shown in Figure 2.31 [151, 152]. The results imply that polarized infrared irradiation is a potential technique for alignment of DLC semiconductors: circular polarized one is for homeotropic alignment and linear polarized one is for planar alignment.
Figure 2.29 Synchrotron X-ray diffraction pattern (left) and X-ray intensity versus diffraction angle plot (right) obtained from homeotropic monodomain of sandwiched sample 34 with an 8 µm gap at 126°C. Reproduced with permission from ref. 77(b).
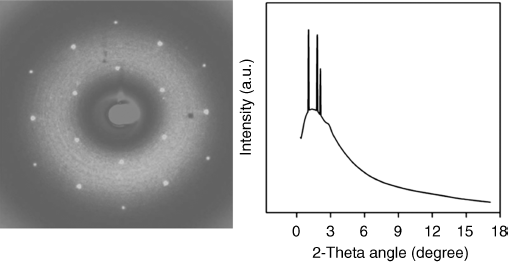
Figure 2.30 Fabrication process of homeotropically aligned thin films of phthalocyanine derivative. The last step serves only to demonstrate the function of the sacrificial layer. Reproduced with permission from ref. 148.
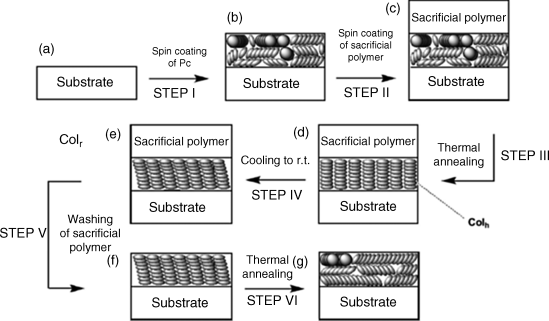
Figure 2.31 (a) Schematic representations of alignment change of 8 with the circularly polarized IR irradiation. Reproduced with permission from ref. 152. Alignment change of 9: (b) infrared irradiation with linearly polarized light and (c) irradiation with circularly polarized light. Reproduced with permission from ref.151.
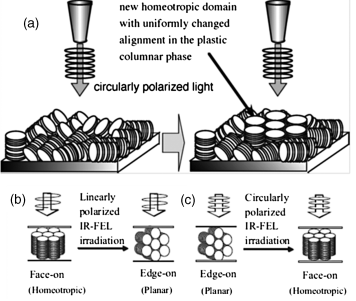
Additionally, Kang et al. have investigated some porphyrin-based DLCs for their alignment behavior which preferred homeotropic alignment. In thinner cells (<10 µm), there was even more uniform homeotropic alignment. The example of compound 33 is shown in Figure 2.32. The control of orientational order of columns was found to be achieved by varying the film thickness, thermal annealing process, and mechanical shearing [77].
Figure 2.32 Formation of homeotropic texture on a macroscopic scale via slow cooling of the 1.8 µm cell with 33. Slow cooling induces selective nucleation and growth of homeotropic domain (parts (a) and (b) at 121.0°C) and hence yields uniform homeotropic columnar orientation at lower temperatures ((c) 113.0°C and (d) 75.0°C), confirmed by both (e) optical texture under crossed polarizers and (f) conoscopic image at 75.0°C. Optical images were taken at (a–d) 70° and (e) 90° angles between polarizers. The scale bar corresponds to 50 µm. (g) X-ray 2D pattern for the Colh phase at 75.0°C of 33. (h) The azimuthal scan of the peak in (g) shows equally spaced six peaks with uniform intensity distribution. Reproduced with permission from ref. 77(a). (See the color version of this figure in Color Plates section.)
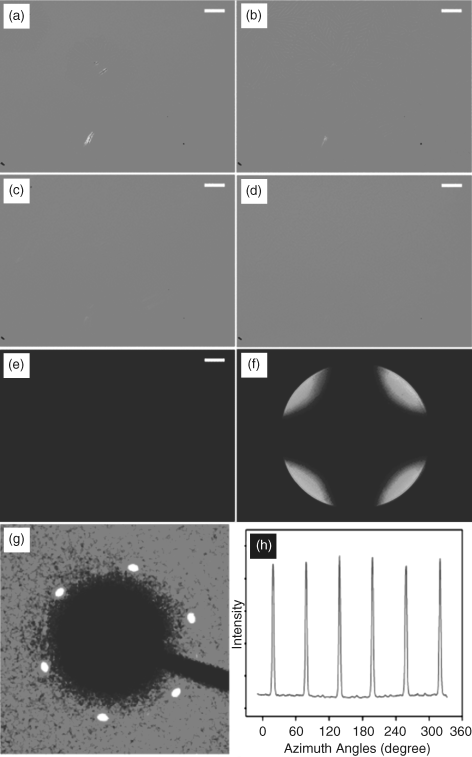
2.4.2 Planar Alignment
Discotic columns with planar alignment are very important for applications in OFETs. To improve device performance, a well-ordered and uniaxially aligned thin layer of discotic columns exhibiting high charge-carrier mobility is required. Technically, to achieve planar alignment of organic discotic semiconducting molecules, various approaches such as applying aligned polytetrafluoroethylene (PTFE) layers, zone casting, Langmuir–Blodgett (LB) films, self-assembled monolayers (SAM), pulsed infrared irradiation, and magnetic field have been developed. Among these methods, while some of them are employed for solid state materials, the use of an aligned PTFE layer and zone casting offers a way for solution samples: by spin coating the material onto an aligned PTFE layer, Zimmermann et al. first reported the “edge-on” orientation of a triphenylene compound in its hexagonal plastic columnar phase [153]. Furthermore, PTFE-coated surfaces were later applied for aligning other materials like HBCs. Friend et al. reported planar alignment of an HBC derivative on rubbed-PTFE layer and obtained field-effect mobility as high as 0.5 × 10−3 cm2 V−1 s−1, even without an annealing step [154]. With zone-casting (Figure 2.33) films of discotic HBC 61, Müllen et al. reported higher FET device performance (on-off ratio of 104 and a field-effect mobility of ~5 × 10−3 cm2 V−1 s−1) [155, 156].
Figure 2.33 Schematic presentation of the zone-casting technique on 61 displaying homogeneous film formation lying in the zone-casting direction. Reproduced with permission from ref. 155.
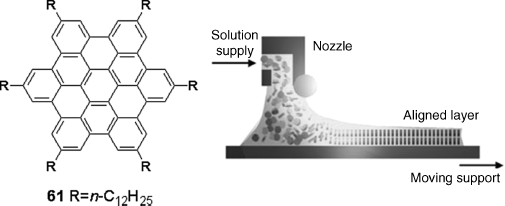
Using the Langmuir–Blodgett film technique is another frequently applied method for fabricating ultrathin ordered layers of discotic molecules. In this technique, amphiphilic molecules can “edge on” align at the air–water interface, which is thereafter effectively transferred to a solid substrate by vertical dipping and raising of the substrate (Figure 2.34) [157]. When spread at the air–water interface, DLC materials such as triphenylenes, phthalocyanines, and HBCs that have been partially functionalized with hydrophilic side chains are reported to form well-ordered Langmuir monolayers [158–160].
Figure 2.34 The Langmuir–Blodgett technique. Left side: A, deposition of the amphiphiles on the water subphase with a solid substrate sub-merged; B, by action of the barrier the monolayer is compressed; C, by pulling out the substrate vertically a monolayer is transferred to both sides of the substrate; D, a multilayered structure is built up by repeated up and down strokes of the substrate; E, transfer of monolayer by horizontal lifting (Schaefer method). Right side: examples of different types of multilayer structures (X, Y, and Z) which can be built up by the LB technique. Reproduced with permission from ref. 157.
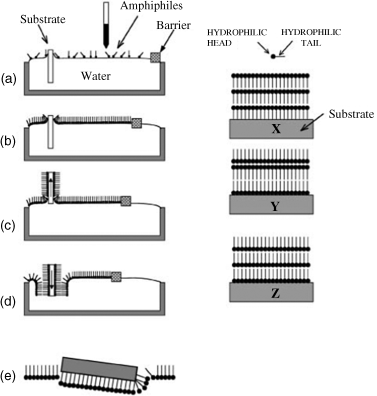
For other approaches, Räder et al. have reported a soft-landing method in which matrix-assisted laser desorption/ionization (MALDI) mass spectrometry can produce ordered structures of organic macromolecules at surfaces [161]. Application of a strong magnetic field (20 T) was used to produce large-area monodomain films of discotic HBC molecules, by which constructed solution-processed FETs exhibited charge-carrier mobilities up to 10−3 cm2 V−1 s−1, significantly higher than that of unaligned material [162]. A. Calo et al. demonstrated a viable method to yield highly oriented DLC films by controlling the entangled phenomena of dewetting and electromigration in DLC films. This approach provides a powerful tool for aligning DLC in a bottom-electrode device channel which is potentially useful for stabilizing and optimizing the device performance of other classes of mesogenic organic semiconductors in ultrathin film devices [163].
Recently, there have been extensive reports of DLC materials with potentially attractive properties. Development of a suitable processing technique aligning DLCs in the highly ordered arrangement is critical in optimizing their desirable properties in practical electronic devices. This is an urgent task that encourages researchers recently and it is expected that DLCs will have an excellent performance in opto-electronics in the near future.
2.5 Applications of Self-Assembled DLCs
Although practical useful discotic nematic liquid crystals have been used in commercial applications for the development of an optical compensator film for improving the view angle of liquid crystal displays (LCDs) [2, 3, 164], there has been a rapidly growing research interest in the recent decade for non-display applications in various molecular electronic devices, such as solar cells, OLEDs and OFETs.
2.5.1 Solar Cells
Solar cells (also known as photovoltaic cells) allow the conversion of light energy into electrical energy. During the past years, organic photovoltaic cells consisting of semiconducting thin films sandwiched between two electrodes have been developed. A model is shown in Figure 2.35. Unlike inorganic semiconductors where free electron hole pairs are photoinduced, organic semiconductors generate excitons (bound hole–electron pairs) by photoabsorption. After generation, the excitons move by hopping between neighboring sites. The transport efficiency is limited by the diffusion length (the distance over which the excitons can travel without recombination). For organic semiconductors, exciton diffusion lengths are usually of 10–20 nm. The performance of a solar cell device critically depends on several factors such as absorption of incident light, exciton diffusion length, charge separation, and charge collection at the electrodes [165]. In solar cells, the UV–Visible absorption capability of the materials is very important, as it directly relates to the quantity of photons that the device can capture from the sun to form excitons. Most organic semiconducting materials possess a high absorption coefficient (α > 105 cm−1), meaning thin layer can work efficiently for absorbing light. Equally important are the relative energy levels of the donor and acceptor materials because the energy gap between HOMO of the donor and LUMO of the acceptor determines the efficient charge separation at the interface of donor–acceptor, as well as the potential output (open-circuit voltage) of the device. Once an exciton reaches the donor–acceptor interface, a polaron pair forms: the electron promoted in the LUMO level of the excited donor transfer to the lower LUMO level of the acceptor, with the hole remaining in the HOMO level of the donor. Generally in organic semiconducting materials, by overcoming the intrinsic exciton-binding energy, charge separation will occur at the donor–acceptor interface and this is the major source of free charges: electrons and holes. Furthermore, to generate current the dissociated electrons and holes have to move to their corresponding electrodes under a built-in electric potential created by connecting two electrodes. In such a device, when electrons flow from the low-work-function electrode to the high-work-function electrode for equalizing the Fermi energies of the two electrodes, a built-in electric field forms. Because this built-in electric field can pull the photo-induced charge carriers (electrons and holes) to their corresponding electrodes, a current and a voltage may appear. For improving energy conversion efficiency, single layer, bilayer, and bulk heterojunction (BHJ) cells were developed one by one in history (Figure 2.35). In the case of heterojunction devices, two organic semiconducting materials with different electron affinities (LUMO) or ionization potentials (HOMO) are combined together to provide sufficient contact area.
Figure 2.35 Top: Schematic diagram of an organic solar cell (heterojunction) (left) and the corresponding energy-level diagram showing charge separation and transport (right). Bottom: Three types of organic layer structures.
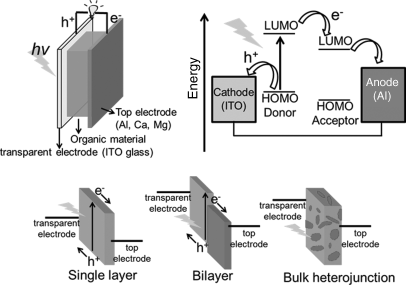
Discotic columns as an active semiconducting layer in OPV cells have a number of advantages over other organic materials. Apart from the easy solution and/or melt processing into large-area thin films on flexible substrates, the highly ordered columnar mesophase of DLCs offers large exciton diffusion length and high charge-carrier mobilities, which are both key parameters for the device efficiency. Moreover, the large π-conjugated aromatic cores of DLCs can be particularly attractive because of their absorption capabilities over the entire solar spectrum. Calculations based on the absorption coefficient of organic materials reveal that a film thickness of 50–100 nm is often required to absorb up most of the incident light. Experiments have shown that an exciton can diffuse only around 5–40 nm in most organic semiconductors before recombination. Highly ordered homeotropically aligned DLC films support efficient transport of charge carriers in relatively thick films (up to a few 100 nm), preventing the possible recombination during their journey to the electrodes. Thus, the high intrinsic charge-carrier mobilities reported for several discotic materials have highlighted their potentials for solar cell applications.
Good discotic materials for photovoltaic applications should have certain prerequisites such as (i) high absorption over the wavelengths of the solar spectrum, (ii) exhibiting spontaneous homeotropic alignment in their columnar mesophase, and (iii) energy levels matching well with the electron acceptors and anode materials to facilitate charge separation and transport. Among the most promising columnar discotics that have been investigated so far for solar cell applications, there are DLC materials such as porphyrin, phthalocyanine, HBC, triphenylene, and perylene derivatives. The first DLC film having a photovoltaic effect was published in 1990 by Gregg et al., who sandwiched a porphyrin DLC layer between ITO-coated glass electrodes [166]. Years later, Petritsch et al. reported photovoltaic performance in a bilayer cell composed of a DLC donor–acceptor pair: a phthalocyanine derivative as an electron donor and a perylene derivative as an electron acceptor [167]. A milestone improvement in photovoltaic performance by combining a p-type discotic HBC molecule (hexaphenyl-substituted hexabenzocoronene) with an n-type perylene molecule (N,N′-bis(1-ethylpropyl)-3,4,9,10-perylenebis dicarboximide) in a BHJ device, achieving a maximum external quantum efficiency of more than 34% and a power conversion efficiency around 2% under monochromatic illumination of 490 nm was reported by Schmidt-Mende et al. [143]. It is due to the self-assembled DLC HBC and perylene compounds stacking along the π-system that the high efficiency of photoinduced charge transfer can be achieved. Recently, bilayer and BHJ solar cells by solution processing of discotic porphyrin donors with C60 or PC61BM acceptors were fabricated [78, 79]. In Figure 2.36, the BHJ PV cell formed from a 1:1 (w/w) blend of a DLC porphyrin electron donor (31) and PC61BM electron acceptor presented a power conversion efficiency (PCE) of 0.22%, where the ITO anode was coated with a conducting polymer poly(3,4-ethylenedioxythiophene) (PEDOT) and the cathode was Ca/Al. Upon post annealing, which lead to a better alignment of the porphyrin, the PCE increased to 0.712%.
Figure 2.36 J–V characteristics of the bulk heterojunction cell. (a) Time dependences of the induced currents in normalized current density per 1 mm2 area in 3 homeotropically aligned thin-film cells (g-i: gold–ITO; i-i: ITO–ITO electrode pairs). The light source was a mercury lamp. The area of the cell was measured directly. (b) J–V characteristics of the bulk-heterojunction solar cells based on 3 (a: in the dark; b: under illumination of AM 1.5 G, 100 mW cm−2; c: cooling to room temperature after heating the cell to 145°C, then under illumination of AM 1.5 G, 100 mW cm−2). Reproduced with permission from ref. 78.
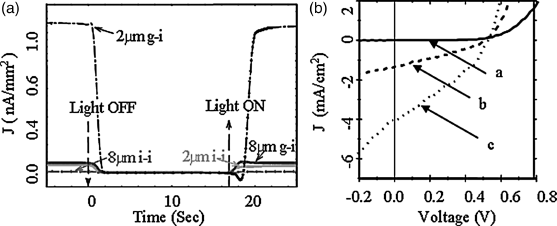
2.5.2 OLEDs
The reverse of a solar cell configuration is the OLED device. Generally, OLEDs generate light by electroluminescence. The basic structure of an OLED is presented in Figure 2.37.
Figure 2.37 Schematic diagram of an OLED device. Electrons introduced at the metal electrode (cathode) recombine with holes introduced at the ITO electrode (anode) to emit light.
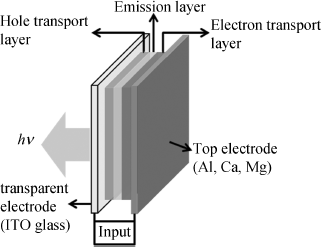
Sandwiched between two electrodes lies a thin film of stacked organic semiconductors, consisting of three sub-layers: a hole transport layer, an emission layer, and an electron transport layer. In many cases, an electron-transporting layer and a light-emitting layer are combined together into one layer. On one side, the low-work-function electrode such as Al or Mg injects electrons into the LUMO of the electron-transporting material. On the other side, a thin film of the transparent ITO deposited onto the substrate usually serves as the anode which injects holes into the HOMO of the hole-transporting material. Under a voltage across the OLED, electrons and holes transport through their corresponding layers and recombine at the interface (emission layer), and then light is emitted.
The key properties for OLEDs are efficient charge transport and light emission. As excellent charge transport was evidenced in the columnar mesophases, DLC materials have caught attention for OLED application. After Wendorff et al. first pointed out the potential use of columnar discotics for applications in single-layer OLEDs, shortly Bacher et al. reported bilayer OLEDs composing low-molar-mass polymeric discotic triphenylene compounds [168–170].
For typical OLED bilayer example, in Figure 2.38(a) a red organic light emitting device made from DLC materials, the p-type 2,3,6,7,10,11-hexabutoxytriphenylene and n-type fluorescent tetraethylperylene-3,4,9,10-tetracarboxylate was reported by Nguyen et al. [171]. The typical OLED device structure is composed of ITO/triphenylene hexaether/perylene tetraester/aluminium. Electrons are injected from the aluminium cathode into the perylene layer and holes are injected from the ITO anode into the triphenylene layer. Then the charge recombination occurs in the perylene layer close to the heterojunction, emitting a red light (615 nm). In Figure 2.38(b), the current density was discovered to reach the maximum of 28 mA cm−1 at 26 V, while at 28 V a luminance maximum as 45 cd m−1 was reported when the light was turned on at 16 V. Furthermore, Destruel et al. reported red, green, and blue light by combining highly fluorescent and electron-deficient discotic perylene derivative with an electron-rich triphenylene (as hole transport material) in a bilayer/multilayer OLED devices [66, 172].
Figure 2.38 (a) Band diagram of OLED made of stacked triphenylene and perylene layers. (b) Current density–voltage characteristics of the electron-luminescence device. Reproduced with permission from ref. 171.
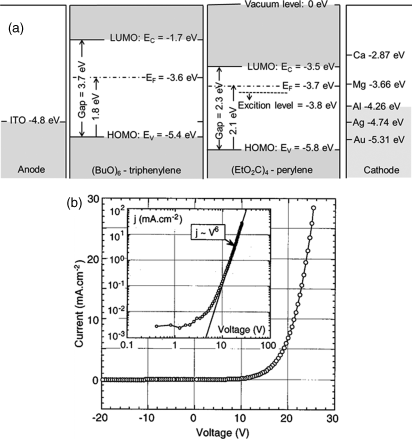
2.5.3 OFETs
In FET devices controlled by an electric field, the current can flow along a semiconductor path (channel). Electrons can move along this path by continually putting electrons in one end (the source electrode) and moving out at the other (the drain electrode). An OFET can be fabricated in either top- or bottom-contact configuration (Figure 2.39). In top-contact form, the drain and source electrodes are deposited on top of the semiconducting layer, while in bottom-contact form the electrodes are positioned under the active layer. By inputting a voltage through a control electrode called the gate, which is often a highly doped silicon substrate, a large variation in the current flow from the source to the drain can be achieved. In a typical OFET device, a thin organic semiconducting film forms ohmic contacts (negligible resistance) to the drain and source electrodes. For the electrodes, commonly Au is used and vacuum deposition is used for fabrication. If there is no gate voltage applied, the organic semiconductor, which is intrinsically undoped, will not show any charge carriers.
Figure 2.39 OFET device configurations: (a) Top-contact device, with source and drain electrodes deposited onto the organic semiconducting layer. (b) Bottom-contact device, with organic semiconductor deposited onto prefabricated source and drain electrodes.
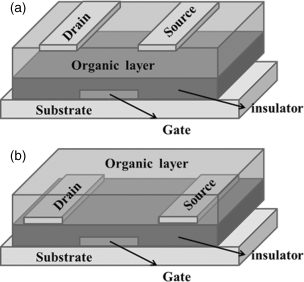
Here is an example of a typical OFET device based on the zone-casting technique. Figure 2.40 shows an OFET device constructed using aligned 61 with top contacts of 25 µm channel length which exhibits saturation mobilities up to 5 × 10−3 cm2 V−1 s−1 [155]. The on-off ratio was 104 and the turn-on voltage was ~15 V.
Figure 2.40 (a) Schematic presentation of the top-contact device configuration (channel length L = 25 mm, width W = 1.6 mm, and depth d = 200 nm) onto a 20 nm thick zone-cast 62 aligned film. A 200 nm SiO2 dielectric gate and an n-doped silicon substrate as a gate electrode were used in this device configuration. (b) Transfer characteristics (gate voltage Vg = −40 V). (c) Current–voltage (I–V) output characteristics. Both dependences are measured along the columnar alignment. Vd: drain voltage; Isd: source–drain current. Reproduced with permission from ref. 155.
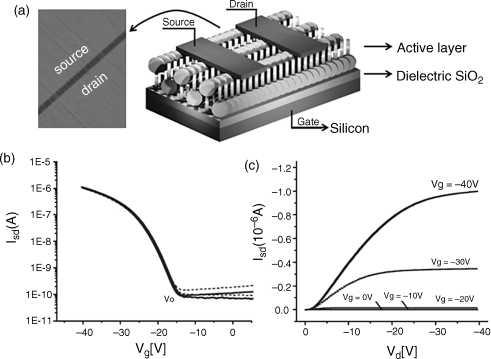
When a voltage is applied to the gate electrode, a thin layer of accumulated charge at the semiconductor–dielectric interface forms, resulting in a current flow between source and drain electrodes. They can be potentially used for thin film transistor applications in active matrix displays, sensors, smart cards, and radiofrequency identification tags [173].
In recent years, dramatic improvement of OFETs has been achieved and high-performance DLC materials for OFET applications have been demonstrated, such as derivatives of HBCs, phthalocyanines, and perylenes [174]. Armstrong et al. fabricated bottom-contact transistors based on a discotic Cu-phthalocyanine derivative on silicon substrates with the LB alignment technique, showing a field mobility value of 10−2 cm2 V−1 s−1 [175]. Much higher field-effect mobility (as high as 2.1 cm2 V−1 s−1) with perylene diimide 19c by annealing at an adequate temperature (140°C) after device fabrication can be achieved [176]. One significant drawback of semiconducting perylene diimide derivatives is that they lack stability under ambient conditions in air. However, this problem can be fixed by introducing substitution of fluoroalkyl chains in the imide substituents which improves the air stability of perylene diimide organic semiconductors [177]. For more information about applications of DLC materials, see reference [178].
2.6 Conclusions and Outlook
In this chapter, the principles of DLC materials have been comprehensively introduced. When focusing on the columnar phases of DLCs, it has become evident that the electronic delocalization of intracolumn molecules has introduced prominent features: (1) low band gap and low reorganization energies; (2) large exciton diffusion length; and (3) high charge carrier mobility. Until now the research activities on these materials have been primarily focused on synthesis and processing of new materials. The overall objectives of the research on DLCs are to achieve the following purposes: (1) introducing functionalities through various molecular structures and supramolecular assemblies; (2) processing alignments to satisfy performance requirements in devices; and (3) increasing order of defect-free DLC mesophase to improve charge transport. In recent years, other impressive achievements have been made. A number of perspectives of new and exciting research are being explored, for example, the control of supramolecular order, the miscibility and the morphology of discotic blends, the use of helical columnar structures for generating large non-linear optical responses, the combination of charge transport with magnetic properties, and the incorporation of columnar structures in membranes.
In summary, the self-organized DLCs are very powerful functional materials for many applications, particularly for energy production and charge migration purposes. Publications and patents of recent decades indicate that there will be a continuous tremendous interest in creating a new generation of organic semiconducting DLCs. For future investigations, there will be considerable efforts to uncover the full potential of discotic semiconductors for not only improving their device performances, but also bringing them to the consumer market for the benefit of societal needs.
Acknowledgments
The preparation of this chapter benefited from the support to Quan Li by the Ohio Board of Regents under its Research Challenge program, the Department of Energy (DOE DE-SC0001412), the Air Force Office of Scientific Research (AFOSR FA9550-09-1-0193 and FA9550-09-1-0254), the Department of Defense Multidisciplinary University Research Initiative (AFOSR MURI FA9550-06-1-0337 and FA9550-12-1-0037), and the National Science Foundation (NSF IIP 0750379).
1. S. Chandrasekhar, B. K. Sadashiva, and K. A. Suresh. Liquid crystals of disk-like molecules. Pramana 1977, 7, 471–480.
2. S. Kumar and S. K. Varshney. A room-temperature discotic nematic liquid crystal. Angew. Chem. Int. Ed. 2000, 39, 3140–3142.
3. M. Okazaki, K. Kawata, H. Nishikawa, and M. Negoro. Polymerizable discotic nematic triphenylene derivatives and their application to an optically anisotropic film. Polym. Adv. Technol. 2000, 11, 398–403.
4. V. de Cupere, J. Tant, P. Viville, R. Lazzaroni, W. Osikowicz, W. R. Salaneck, and Y. H. Geerts. Effect of interfaces on the alignment of a discotic liquid−crystalline phthalocyanine. Langmuir 2006, 22, 7798–7806.
5. A. J. J. M. van Breemen, P. T. Herwig, C. H. T. Chlon, J. Sweelssen, H. F. M. Schoo, S. Setayesh, W. M. Hardeman, C. A. Martin, D. M. de Leeuw, J. J. P. Valeton, C. W. M. Bastiaansen, D. J. Broer, A. R. Popa-Merticaru, and S. C. J. Meskers. Large area liquid crystal monodomain field-effect transistors. J. Am. Chem. Soc. 2006, 128, 2336–2345.
6. D. Demus. One century liquid crystal chemistry: from Vorlander's rods to disks, stars and dendrites. Mol. Cryst. Liq. Cryst. 2001, 364, 25–91.
7. S. Kumar. Self-organization of disc-like molecules: chemical aspects. Chem. Soc. Rev. 2006, 35, 83–109.
8. C. Tschierske. Micro-segregation, molecular shape and molecular topology partners for the design of liquid crystalline materials with complex mesophase morphologies. J. Mater. Chem. 2001, 11, 2647–2671.
9. C. Tschierske. Liquid crystalline materials with complex mesophase morphologies. Curr. Opin. Colloid Interface Sci. 2002, 7, 69–80.
10. X. Crispin, J. Cornil, R. Friedlein, K. K. Okudaira, V. Lemaur, A. Crispin, G. Kestemont, M. Lehmann, M. Fahlman, R. Lazzaroni, Y. Geerts, G. Wendin, N. Ueno, J.-L. Bredas, and W. R. Salaneck. Electronic delocalization in discotic liquid crystals: a joint experimental and theoretical study. J. Am. Chem. Soc. 2004, 126, 11889–11899.
11. J. M. Warman, M. P. de Haas, G. Dicker, F. C. Grozema, J. Piris, and M. G. Debije. Charge mobilities in organic semiconducting materials determined by pulse-radiolysis time-resolved microwave conductivity: π-bond-conjugated polymers versus π−π stacked discotics. Chem. Mater. 2004, 16, 4600–4609.
12. Z. An, J. Yu, S. C. Jones, S. Barlow, S. Yoo, B. Domercq, P. Prins, L. D. A. Siebbeles, B. Kippelen, and S. R. Marder. High electron mobility in room-temperature discotic liquid-crystalline perylene diimides. Adv. Mater. 2005, 17, 2580–2583.
13. B. A. Jones, M. J. Ahrens, M.-H. Yoon, A. Facchetti, T. J. Marks, and M. R. Wasielewski. High-mobility air-stable n-type semiconductors with processing versatility: dicyanoperylene-3,4:9, 10-bis(dicarboximides). Angew. Chem. Int. Ed. 2004, 43, 6363–6366.
14. H. Iino, Y. Takayashiki, J.-I. Hanna, and R. J. Bushby. Fast ambipolar carrier transport and easy homeotropic alignment in a metal-free phthalocyanine derivative. Jpn. J. Appl. Phys. Part 2 2005, 44, 1310–1312.
15. D. Markovitsi, S. Marguet, J. Bondkowski, and S. Kumar. Triplet excitation transfer in triphenylene columnar phases. J. Phys. Chem. B 2001, 105, 1299–1306.
16. N. Boden, R. J. Bushby, J. Clements, B. Movaghar, K. J. Donovan, and T. Kreouzis. Mechanism of charge transport in discotic liquid crystals. Phys. Rev. B 1995, 52, 13274–13279.
17. K. Kohary, H. Cordes, S. D. Baranovskii, P. Thomas, S. Yamasaki, F. Hensel, and J.-H. Wendorff. One-dimensional hopping transport in disordered organic solids. II. Monte Carlo simulations. Phys. Rev. B 2001, 63, 094202.
18. L. J. Lever, R. W. Kelsall, and R. J. Bushby. Band transport for discotic liquid crystals. Phys. Rev. B 2005, 72, 035130.
19. J. Zaumseil and H. Sirringhaus. Electron and ambipolar transport in organic field-effect transistors. Chem. Rev. 2007, 107, 1296–1323.
20. R. G. Kepler. Charge carrier production and mobility in anthracene crystals. Phys. Rev. 1960, 119, 1226–1229.
21. O. H. Leblanc. Hole and electron drift mobilities in anthracene. J. Chem. Phys. 1960, 33, 626–627.
22. D. Adam, F. Closs, T. Frey, D. Funhoff, D. Haarer, H. Ringsdorf, P. Schuhmacher, and K. Siemensmeyer. Transient photoconductivity in a discotic liquid crystal. Phys. Rev. Lett. 1993, 70, 457–460.
23. A. M. Van de Craats, J. M. Warman, M. P. De Haas, D. Adam, J. Simmerer, D. Haarer, and P. Schuhmacher. The mobility of charge carriers in all four phases of the columnar discotic material hexakis (hexylthio) triphenylene: combined TOF and PR-TRMC results. Adv. Mater. 1996, 8, 823–826.
24. W. Pisula, M. Zorn, J. Y. Chang, K. Müllen, and R. Zental. Liquid crystalline ordering and charge transport in semiconducting materials. Macromol. Rapid Commun. 2009, 30, 1179–1202.
25. J. M. Warman and A. M. Van de Craats. Charge mobility in discotic materials studied by PR-TRMC. Mol. Cryst. Liq. Cryst. 2003, 396, 41–72.
26. A. M. van de Craats and J. M. Warman. The core-size effect on the mobility of charge in discotic liquid crystalline materials. Adv. Mater. 2001, 13, 130–133.
27. J. M. Warman, M. P. de Haas, G. Dicker, F. C. Grozema, J. Piris, and M. G. Debije. Charge mobilities in organic semiconducting materials determined by pulse-radiolysis time-resolved microwave conductivity: π-bond-conjugated polymers versus π–π stacked discotics. Chem. Mater. 2004, 16, 4600–4609.
28. A. M. Van de Craats, J. M. Warman, A. Fechtenkötter, J. D. Brand, M. A. Harbison, and K. Müllen. Record charge carrier mobility in a room-temperature discotic liquid crystalline derivative of hexabenzocoronene. Adv. Mater. 1999, 11, 1469–1472.
29. P. W. M. Blom, M. J. M. deJong, and J. J. M. Vleggaar. Electron and hole transport in poly(p-phenylene vinylene) devices. Appl. Phys. Lett. 1996, 68, 3308–3310.
30. D. Fichou. Handbook of Oligo- and Polythiophenes, Wiley-VCH, Weinheim, New York, 1999.
31. G. Horowitz. Organic field-effect transistors. Adv. Mater. 1998, 10, 365–377.
32. G. Horowitz. Organic thin film transistors: from theory to real devices. J. Mater. Res. 2004, 19, 1946–1962.
33. A. Dodabalapur, L. Torsi, and H. E. Katz. Organic transistors: two-dimensional transport and improved electrical characteristics. Science 1995, 268, 270–271.
34. P. V. Pesavento, R. J. Chesterfield, C. R. Newman, and C. D. Frisbie. Gated four-probe measurements on pentacene thin-film transistors: contact resistance as a function of gate voltage and temperature. J. Appl. Phys. 2004, 96, 7312–7324.
35. C. Goldmann, S. Haas, C. Krellner, J. P. Pernstich, D. J. Gundlach, and B. Batlogg. Hole mobility in organic single crystals measured by a “flip-crystal” field-effect technique. J. Appl. Phys. 2004, 96, 2080–2086.
36. V. Podzorov, S. E. Sysoev, E. Loginova, V. M. Pudalov, and M. E. Gershenson. Single-crystal organic field effect transistors with the hole mobility ~8 cm2/V s. Appl. Phys. Lett. 2003, 83, 3504–3506.
37. A. F. Stassen, R. W. I. de Boer, N. N. Iosad, and A. F. Morpurgo. Influence of the gate dielectric on the mobility of rubrene single-crystal field-effect transistors. Appl. Phys. Lett. 2004, 85, 3899–3901.
38. J. Veres, S. D. Ogier, S. W. Leeming, D. C. Cupertino, and S. M. Khaffaf. Low-k insulators as the choice of dielectrics in organic field-effect transistors. Adv. Funct. Mater. 2003, 13, 199–204.
39. B. Domercq, J. Yu, B. R. Kaafarani, T. Kondo, S. Yoo, J. N. Haddock, S. Barlow, S. R. Marder, and B. Kippelen. A comparative study of charge mobility measurements in a diamine and in a hexaazatrinaphthylene using different techniques, Mol. Cryst. Liq. Cryst. 2008, 481, 80–93.
40. A. Troisi, D. L. Cheung, and D. Andrienko. Charge transport in semiconductors with multiscale conformational dynamics. Phys. Rev. Lett. 2009, 102, 116602.
41. F. C. Grozema and L. D. A. Siebbeles. Mechanism of charge transport in self-organizing organic materials. Int. Rev. Phys. Chem. 2008, 27, 87–138.
42. J. Y. Chang, J. R. Yeon, Y. S. Shin, M. J. Han, and S. K. Hong. Synthesis and characterization of mesogenic disk-like benzenetricarboxylates containing diacetylenic groups and their polymerization. Chem. Mater. 2000, 12, 1076–1082.
43. A. N. Cammidge and H. Gopee. Macrodiscotic triphenylenophthalocyanines. Chem. Commun. 2002, 966–967.
44. A. Hayer, V. de Halleux, A. Koehler, A. El-Garoughy, E. W. Meijer, J. Barbera, J. Tant, J. Levin, M. Lehmann, J. Gierschner, J. Cornil, and Y. H. Geerts. Highly fluorescent crystalline and liquid crystalline columnar phases of pyrene-based structures. J. Phys. Chem. B 2006, 110, 7653–7659.
45. J. E. Kroeze, R. B. M. Koehorst, and T. J. Savenije. Singlet and triplet exciton diffusion in a self-organizing porphyrin antenna layer. Adv. Funct. Mater.2004,14, 992–998; H. Eichhorn. Mesomorphic phthalocyanines, tetraazaporphyrins, porphyrins and triphenylenes as charge-transporting materials. J. Porphyrin Phthalocyanines 2000, 4, 88–102.
46. B. Cabezon, M. Nicolau, J. Barbera, and T. Torres. Synthesis and liquid-crystal behavior of triazolephthalocyanines. Chem. Mater.2000,12, 776–781; J. F. van der Pol, E. Neeleman, J. W. Zwikker, R. J. M. Nolte, W. Drenth, J. Aerts, R. Visser, and S. J. Picken. Homologous series of liquid-crystalline metal free and copper octa-n-alkoxyphthalocyanines. Liq. Cryst.1989,6, 577–592; K. Ohta, L. Jacquemin, C. Sirlin, L. Bosio, and J. Simon. Influence of the nature of the side chains on the mesomorphic properties of octasubstituted phthalocyanine derivatives. New J. Chem.1988,12, 751–754; D. Guillon, A. Skoulios, C. Piechocki, J. Simon, and P. Weber. The crystal and molecular structure of a reentrant nematogen: 4-cyanophenyl-3-methyl-4′-(4″-n-undecyloxy cinnamoyloxy) benzoate (11 CPMCB). Mol. Cryst. Liq. Cryst.1983,100, 275–284; L. Dulog and A. Gittinger. Octaalkyl esters of 2,3,9,10,16,17,23,24-(29H, 31H)-phthalocyanineoctacarboxylic acid: a new homologous series of discotic liquid crystals. Mol. Cryst. Liq. Cryst.1992,213, 31–42; S. Sergeyev, E. Pouzet, O. Debever, J. Levin, J. Gierschner, J. Cornil, R. Gomez-Aspe, and Y. H. Geerts. Liquid crystalline octaalkoxycarbonyl phthalocyanines: design, synthesis, electronic structure, self-aggregation and mesomorphism. J. Mater. Chem. 2007, 17, 1777–1784.
47. J. Wu, M. D. Watson, L. Zhang, Z. Wang, and K. Müllen. Hexakis(4-iodophenyl)-peri-hexabenzocoronene: a versatile building block for highly ordered discotic liquid crystalline materials. J. Am. Chem. Soc. 2004, 126, 177–186.
48. A. N. Cammidge and R. J. Bushby.In: D. Demus, J. W. Goodby, G. W. Gray, H. W. Spiess, and V. Vill,Eds., Handbook of Liquid Crystals, vol. 2B, Wiley-WCH, Weinheim, 1998.
49. C. Piechocki, J. Simon, A. Skoulios, D. Guillon, and P. Weber. Discotic mesophases obtained from substituted metallophthalocyanines. Toward liquid crystalline one dimensional conductors. J. Am. Chem. Soc. 1982, 104, 5245–5247.
50. D. Adam, P. Schuhmacher, J. Simmerer, L. Haussling, K. Siemensmeyer, K. H. Etzbach, H. Ringsdorf, and D. Haarer. Fast photoconduction in the highly ordered columnar phase of a discotic liquid crystal. Nature 1994, 371, 141–143.
51. J. A. Rego, S. Kumar, and H. Ringsdorf. Synthesis and characterization of fluorescent, low-symmetry triphenylene discotic liquid crystals: tailoring of mesomorphic and optical properties. Chem. Mater. 1996, 8, 1402–1409.
52. A. Kettner and J. H. Wendorff. Modifications of the mesophase formation of discotic triphenylene compounds by substituents. Liq. Cryst. 1999, 26, 483–487.
53. J. Simmerer, B. Glusen, W. Paulus, A. Kettner, P. Schuhmacher, D. Adam, K.-H. Etzbach, K. Siemensmeyer, J. H. Wendorff, H. Ringsdorf, and D. Haarer. Transient photoconductivity in a discotic hexagonal plastic crystal. Adv. Mater. 1996, 8, 815–819.
54. H. Bengs, F. Closs, T. Frey, D. Funhoff, H. Ringsdorf, and K. Siemensmeyer. Highly photoconductive discotic liquid crystals: structure–property relations in the homologous series of hexa-alkoxytriphenylenes. Liq. Cryst. 1993, 15, 565–574.
55. H. Iino, J. Hanna, C. Jager, and D. Haarer. Fast electron transport in discotic columnar phase of triphenylene derivative, hexabutyloxytriphenylene. Mol. Cryst. Liq. Cryst. 2005, 436, 217–224.
56. H. Iino, Y. Takayashiki, J. Hanna, R. J. Bushby, and D. Haarer. High electron mobility of 0.1 cm2 V-1 s-1 in the highly ordered columnar phase of hexahexylthiotriphenylene. Appl. Phys. Lett. 2005, 87, 192105.
57. M. Lehmann, G. Kestemont, R. Gomez Aspe, C. Buess-Herman, M. H. J. Koch, M. G. Debije, J. Piris, M. P. de Haas, J. M. Warman, and M. D. Watson. High charge-carrier mobility in p-deficient discotic mesogens: design and structure–property relationship. Chem. Eur. J. 2005, 11, 3349–3362.
58. K. Pieterse, P. A. van Hal, R. Kleppinger, J. A. J. M. Vekemans, R. A. J. Janssen, and E. W. Meijer. An electron-deficient discotic liquid-crystalline material. Chem. Mater. 2001, 13, 2675–2679.
59. N. Boden, R. C. Borner, R. J. Bushby, and J. Clements. First observation of an n-doped quasi-one-dimensional electronically-conducting discotic liquid crystal. J. Am. Chem. Soc. 1994, 116, 10807–10808.
60. F. Würthner. Perylene bisimide dyes as versatile building blocks for functional supramolecular architectures. Chem. Commun. 2004, 14, 1564–1579.
61. H. Langhals. Control of the interactions in multichromophores: novel concepts. Perylene bis-imides as components for larger functional units. Helv. Chim. Acta. 2005, 88, 1309–1343.
62. P. R. L. Malenfant, C. D. Dimitrakopoulos, J. D. Gelorme, L. L. Kosbar, T. O. Graham, A. Curioni, and W. Andreoni. N-type organic thin-film transistor with high field-effect mobility based on a N,N-dialkyl-3,4,9,10-perylene tetracarboxylic diimide derivative. Appl. Phys. Lett. 2002, 80, 2517–2519.
63. C. W. Struijk, A. B. Sieval, J. E. J. Dakhorst, M. van Dijk, P. Kimkes, R. B. M. Koehorst, H. Donker, T. J. Schaafsma, S. J. Picken, and A. M. van de Craats. Liquid crystalline perylene diimides: architecture and charge carrier mobilities. J. Am. Chem. Soc. 2000, 122, 11057–11066.
64. F. Würthner, C. Thalacker, S. Diele, and C. Tschierske. Fluorescent J-type aggregates and thermotropic columnar mesophases of perylene bisimide dyes. Chem. Eur. J. 2001, 7, 2245–2253.
65. Z. Chen, M. G. Debije, T. Debaerdemaeker, P. Osswald, and F. Würthner. Tetrachloro-substituted perylene bisimide dyes as promising n-type organic semiconductors: studies on structural, electrochemical and charge transport properties. Chem. Phys. Chem. 2004, 5, 137–139.
66. I. Seguy, P. Destruel, and H. Bock. An all-columnar bilayer light-emitting diode. Synth. Met. 2000, 111, 15–18.
67. S. Alibert-Fouet, S. Dardel, H. Bock, M. Oukachmih, S. Archambeau, I. Seguy, P. Jolinat, and P. Destruel. Electroluminescent diodes from complementary discotic benzoperylenes. Chem. Phys. Chem. 2003, 4, 983–985.
68. F. Nolde, W. Pisula, S. Müller, C. Kohl, and K. Müllen. Synthesis and self-organization of core-extended perylene tetracarboxdiimides with branched alkyl substituents. Chem. Mater. 2006, 18, 3715–3725.
69. J. W. Goodby, P. S. Robinson, B.-K. Teo, and P. E. Cladi. The discotic phase of uroporphyrin octa-N-dodecyl ester. Mol. Cryst. Liq. Cryst. 1980, 56, 303–309.
70. C. Y. Liu and A. J. Bard. Optoelectronic properties and memories based on organic single-crystal thin films. Acc. Chem. Res. 1999, 32, 235–245.
71. Q. D. Zhao, M. Yu, T. F. Xie, L. L. Peng, P. Wang, and D. J. Wang. Photovoltaic properties of a ZnO nanorod array affected by ethanol and liquid-crystalline porphyrin. Nanotechnology 2008, 19, 245706.
72. K. Ohta, N. Ando, and I. Yamamoto. Discotic liquid crystals of transition metal complexes: 22. Synthesis and mesomorphism of octa-alkoxy-substituted tetraphenylporphyrin derivatives and their copper(II) complexes. Liq. Cryst. 1999, 26, 663–668.
73. H. Miwa, N. Kobayashi, K. Ban, and K. Ohta. Synthesis, spectroscopy, electrochemistry, and mesomorphism of triple-decker porphyrins consisting of two cerium ions and three 5, 15-diarylporphyrins. Bull. Chem. Soc. Jpn. 1999, 72, 2719–2728.
74. T. Nakai, K. Ban, K. Ohta, and M. Kimura. Discotic liquid crystals of transition metal complexes. Part 32. 1. Synthesis and liquid-crystalline properties of doubledeckers and tripledeckers based on cerium complexes of bis- and tetrakis(3,4-dialkoxyphenyl)porphyrin. J. Mater. Chem. 2002, 12, 844–850.
75. P. G. Schoten, J. M. Gorman, M. P. deHaas, M. A. Fox, and H. L. Pan. Charge migration in supramolecular stacks of peripherally substituted porphyrins. Nature 1991, 353, 736–737.
76. P. G. Schoten, J. M. Gorman, M. P. deHaas, J. F. van der Pol, and J. W. Zwikker. Radiation-induced conductivity in polymerized and nonpolymerized columnar aggregates of phthalocyanine. J. Am. Chem. Soc. 1992, 114, 9028–9034.
77.(a) S. W. Kang, Q. Li, B. D. Chapman, R. Pindak, J. O. Cross, L. Li, M. Nakata, and S. Kumar. Microfocus X-ray diffraction study of the columnar phase of porphyrin-based mesogens. Chem. Mater.2007,19, 5657-5663; (b) X. Zhou, S. W. Kang, S. Kumar, and Q. Li. Self-assembly of discotic liquid crystal porphyrin into more controllable order nanostructure mediated by fluorophobic effect. Liq. Cryst. 2009, 36, 269–274.
78. L. Li, S. W. Kang, J. Harden, Q. Sun, X. Zhou, L. Dai, A. Jakli, S. Kumar, and Q. Li. Nature-inspired light-harvesting liquid crystalline porphyrins for organic photovoltaics. Liq. Cryst. 2008, 35, 233–239.
79. Q. Sun, L. Dai, X. Zhou, L. Li, and Q. Li. Bilayer-and bulk-heterojunction solar cells using liquid crystalline porphyrins as donors by solution processing. Appl. Phys. Lett. 2007, 91, 253505.
80. B. Tylleman, G. Gbabode, C. Amato, C. Buess-Herman, V. Lemaur, J. Cornil, R. Gómez Aspe, Y. H. Geerts, and S. Sergeyev. Metal-free phthalocyanines bearing eight alkylsulfonyl substituents: design, synthesis, electronic structure, and mesomorphism of new electron-deficient mesogens. Chem. Mater. 2009, 21, 2789–2797.
81. H. Iino, J. Hanna, R. J. Bushby, B. Movaghar, B. J. Whitaker, and M. J. Cook. Very high time-of-flight mobility in the columnar phases of a discotic liquid crystal. Appl. Phys. Lett. 2005, 87, 132102.
82. V. S. Iyer, M. Wehmeier, J. D. Brand, M. A. Keegstra, and K. Müllen. From hexa-peri-hexabenzocoronene to “Superacenes”. Angew. Chem. Int. Ed. 1997, 36, 1604–1607.
83. M. G. Debije, J. Piris, M. P. de Haas, J. M. Warman, Z. Tomovic, C. D. Simpson, M. D. Watson, and K. Müllen. The optical and charge transport properties of discotic materials with large aromatic hydrocarbon cores. J. Am. Chem. Soc. 2004, 126, 4641–4645.
84. M. Kastler, F. Laquai, K. Müllen, and G. Wegner. Room-temperature nondispersive hole transport in a discotic liquid crystal. Appl. Phys. Lett. 2006, 89, 252103.
85. X. Feng, V. Marcon, W. Pisula, M. R. Hansen, J. Kirkpatrick, F. Grozema, D. Andrienko, K. Kremer, and K. Müllen. Towards high charge-carrier mobilities by rational design of the shape and periphery of discotics. Nat. Mater. 2009, 8, 421–426.
86. X. Feng, M. Liu, W. Pisula, M. Takase, J. Li, and K. Müllen. Supramolecular organization and photovoltaics of triangle-shaped discotic graphenes with swallow-tailed alkyl substituents. Adv. Mater. 2008, 20, 2684–2689.
87. S. Xiao, M. Myers, Q. Miao, S. Sanaur, K. Pang, M. L. Steigerwald, and C. Nuckolls. Molecular wires from contorted aromatic compounds. Angew. Chem. Int. Ed. 2005, 44, 7390–7394.
88. Y. Yamamoto, G. Zhang, W. Jin, T. Fukushima, N. Ishii, A. Saeki, S. Seki, S. Tagawa, T. Minari, K. Tsukagoshi, and T. Aida. Ambipolar-transporting coaxial nanotubes with a tailored molecular graphene–fullerene heterojunction. PNAS 2009, 106, 21051–21056.
89. Z. Tomovic, M. D. Watson, and K. Müllen. Superphenalene-based columnar liquid crystals. Angew. Chem. Int. Ed. 2004, 43, 755–758.
90. C. D. Simpson, J. S. Wu, M. D. Watson, and K. Müllen. From graphite molecules to columnar superstructures—an exercise in nanoscience. J. Mater. Chem. 2004, 14, 494–504.
91. M. D. Watson, M. G. Debije, J. M. Warman, and K. Müllen. Peralkylated coronenes via regiospecific hydrogenation of hexa-peri-hexabenzocoronenes. J. Am. Chem. Soc. 2004, 126, 766–771.
92. V. S. Iyer, K. Yoshimura, V. Enkelmann, R. Epsch, J. P. Rabe, and K. Müllen. A soluble C60 graphite segment. Angew. Chem. Int. Ed. 1998, 37, 2696–2699.
93. X. J. Zhang, X. X. Jiang, K. Zhang, L. Mao, J. Luo, C. Y. Chi, H. S. O. Chan, and J. S. Wu. Synthesis, self-assembly, and charge transporting property of contorted tetrabenzocoronenes. J. Org. Chem. 2010, 75, 8069–8077.
94. U. Rohr, C. Kohl, K. Müllen, A. Craats, and J. Warman. Liquid crystalline coronene derivatives. J. Mater. Chem. 2001, 11, 1789–1799.
95. K. Hirota, K. Tajima, and K. Hashimoto. Physicochemical study of discotic liquid crystal decacyclene derivative and utilization in polymer photovoltaic devices. Synth. Met. 2007, 157, 290–296.
96. H. K. Bisoyi and S. Kumar. Room-temperature electron-deficient discotic liquid crystals: facile synthesis and mesophase characterization. New J. Chem. 2008, 32, 1974–1980.
97. Y. F. Bai, K. Q. Zhao, P. Hu, B. Q. Wang, and Y. Shimizu. Synthesis of amide group containing triphenylene derivatives as discotic liquid crystals and organic gelators. Mol. Cryst. Liq. Cryst. 2009, 509, 802–818.
98. R. I. Gearba, M. Lahmann, J. Levin, D. A. Ivanov, M. H. J. Koch, J. Barbera, M. G. Debije, J. Piris, and Y. H. Geerts. Tailoring discotic mesophases: columnar order enforced with hydrogen bonds. Adv. Mater. 2003, 15, 1614–1618.
99. A. Demenev, S. H. Eichhorn, T. Taerum, D. F. Perepichka, S. Patwardhan, F. C. Grozeme, L. D. A. Siebbeles, and R. Klenkler. Quasi temperature independent electron mobility in hexagonal columnar mesophases of an H-bonded benzotristhiophene derivative. Chem. Mater. 2010, 22, 1420–1428.
100. I. Paraschiv, K. de Lange, M. Giesbers, B. van Lagen, F. C. Grozema, R. D. Abellon, L. D. A. Siebbeles, E. J. R. Sudholter, H. Zuilhof, and A. T. M. Marcelis. Hydrogen-bond stabilized columnar discotic benzenetrisamides with pendant triphenylene groups. J. Mater. Chem. 2008, 18, 5475–5481.
101. N. Mizoshita, H. Monobe, M. Inoue, M. Ukon, T. Watanabe, Y. Shimizu, K. Hanabusa, and T. Kato. The positive effect on hole transport behaviour in anisotropic gels consisting of discotic liquid crystals and hydrogen-bonded fibres. Chem. Commun. 2002, 428–429.
102. C. W. Ong, J. Y. Hwang, M. C. Tzeng, S. C. Liao, H. F. Hsu, and T. H. Chang. Dibenzo[a,c]phenazine with six-long alkoxy chains to probe optimization of mesogenic behavior. J. Mater. Chem. 2007, 17, 1785–1790.
103. K. Kishikawa, S. Furusawa, T. Yamaki, S. Kohmoto, M. Yamamoto, and K. Yamaguchi. Novel superstructure of nondiscoid mesogens: uneven-parallel association of half-disk molecules, 3,4,5-trialkoxybenzoic anhydrides, to a columnar structure and its one-directionally geared interdigitation. J. Am. Chem. Soc. 2002, 124, 1597–1605.
104. T. Kato, N. Mizoshita, and K. Kanie. Hydrogen-bonded liquid crystalline materials: supramolecular polymeric assembly and the induction of dynamic function. Macromol. Rapid. Commun. 2001, 22, 797–814.
105. T. Kato. Hydrogen-bonded liquid crystals: molecular self-assembly for dynamically functional materials. In: Molecular self-assembly, a comprehensive summary of mesogenic hydrogen bonded systems. Struct. Bond. 2000, 96, 95–146.
106. M. Hird. Fluorinated liquid crystals—properties and applications. Chem. Soc. Rev. 2007, 36, 2070–2095.
107. N. Terasawa, H. Monobe, K. Kiyohara, and Y. Shimizu. Strong tendency towards homeotropic alignment in a hexagonal columnar mesophase of fluoroalkylated triphenylenes. Chem. Commun. 2003, 1678–1679.
108. B. Alameddine, O. F. Aebischer, W. Amrein, B. Donnio, R. Deschenaux, D. Guillon, C. Savary, D. Scanu, O. Scheidegger, and T. A. Jenny. Mesomorphic hexabenzocoronenes bearing perfluorinated chains. Chem. Mater. 2005, 17, 4798–4807.
109. A. Escosura, M. V. Martínez-Díaz, J. Barberá, and T. Torres. Self-organization of phthalocyanine- [60]fullerene dyads in liquid crystals. J. Org. Chem. 2008, 73, 1475–1480.
110. X. Zhou, S.-W. Kang, S. Kumar, R. R. Kulkarni, S. Z. D. Cheng, and Q. Li. Self-assembly of porphyrin and fullerene supramolecular complex into highly ordered nanostructure by thermal annealing. Chem. Mater. 2008, 20, 3551–3553.
111. Y. H. Geerts, O. Debever, C. Amato, and S. Sergeyev. Synthesis of mesogenic phthalocyanine–C60 donor–acceptor dyads designed for molecular heterojunction photovoltaic devices. Beilstein J. Org. Chem. 2009, 5, 49.
112. L. Sánchez, M. Sierra, N. Martín, A. J. Myles, T. J. Dale, J. Rebek, W. Seitz, and D. M. Guldi. Exceptionally strong electronic communication through hydrogen bonds in porphyrin–C60 pairs. Angew. Chem. Int. Ed. 2006, 45, 4637–4641.
113. H. Imahori, H. Yamada, D. M. Guldi, Y. Endo, A. Shimomura, S. Kundu, K. Yamada, T. Okada, Y. Sakata, and S. Fukuzumi. Comparison of reorganization energies for intra- and inter-molecular electron transfer. Angew. Chem. Int. Ed. 2002, 41, 2344–2347.
114. J. L. Sessler, J. Jayawickramarajah, A. Gouloumis, T. Torres, D. M. Guldi, S. Maldonado, and K. J. Stevenson. Synthesis and photophysics of a porphyrin–fullerene dyad assembled through Watson–Crick hydrogen bonding. Chem. Commun. 2005, 1892–1894.
115. H. Yamada, H. Imahori, Y. Nishimura, I. Yamazaki, T. K. Ahn, S. K. Kim, D. Kim, and S. Fukuzumi. Photovoltaic properties of self-assembled monolayers of porphyrins and porphyrin–fullerene dyads on ITO and gold surfaces. J. Am. Chem. Soc. 2003, 125, 9129–9139.
116. H. Imahori and Y. Sakata. Donor-linked fullerenes: photoinduced electron transfer and its potential application. Adv. Mater. 1997, 9, 537–546.
117. N. Watanabe, N. Kihara, Y. Forusho, T. Takata, Y. Araki, and O. Ito. Photoinduced intrarotaxane electron transfer between zinc porphyrin and fullerene in benzonitrile. Angew. Chem. Int. Ed. 2003, 42, 681–683.
118. H. Imahori, M. E. El-Khouly, M. Fujitsuka, O. Ito, Y. Sakata, and S. Fukuzumi. Solvent dependence of charge separation and charge recombination rates in porphyrin–fullerene dyad. J. Phys. Chem. A 2001, 105, 325–332.
119. T. Torres, A. Gouloumis, D. Sanchez-Garcia, J. Jayawickramarajah, W. Seitz, D. M. Guldi, and J. L. Sessler. Photophysical characterization of a cytidine–guanosine tethered phthalocyanine–fullerene dyad. Chem. Commun. 2007, 292–294.
120. D. W. Bruce, D. A. Dunmur, L. S. Santa, and M. A. Wali. Mesomorphic metalloporphyrins showing calamitic mesophases. J. Mater. Chem. 1992, 2, 363–364.
121. H. N. Tsao, W. Pisula, Z. Liu, W. Osikowicz, W. R. Salaneck, and K. Müllen. From ambi-to unipolar behavior in discotic dye field-effect transistors. Adv. Mater. 2008, 20, 2715–2719.
122. H. Fujikake, T. Murashige, M. Sugibayashi, and K. Ohta. Time-of-flight analysis of charge mobility in a Cu-phthalocyanine-based discotic liquid crystal semiconductor. Appl. Phys. Lett. 2004, 85, 3474.
123. H. Iino, J. Hanna, R. J. Bushby, B. Movaghar, B. J. Whitaker, and M. J. Cook. Very high time-of-flight mobility in the columnar phases of a discotic liquid crystal. Appl. Phys. Lett. 2005, 87, 132102.
124. K. Ban, K. Nishizawa, K. Ohta, A. M. Craats, J. M. Warman, I. Yamamoto, and H. Shirai. Discotic liquid crystals of transition metal complexes 29: mesomorphism and charge transport properties of alkylthio-substituted phthalocyanine rare-earth metal sandwich complexes. J. Mater. Chem. 2001, 11, 321–331.
125. T. Sakurai, K. Shi, H. Sato, K. Tashiro, A. Osuka, A. Saeki, S. Seki, S. Tagawa, S. Sasaki, H. Masunaga, K. Osaka, M. Takata, and T. Aida. Prominent electron transport property observed for triply fused metalloporphyrin dimer: directed columnar liquid crystalline assembly by amphiphilic molecular design. J. Am. Chem. Soc. 2008, 130, 13812–13813.
126. M. Xiao and P. R. Selvin. Quantum yields of luminescent lanthanide chelates and far-red dyes measured by resonance energy transfer. J. Am. Chem. Soc. 2001, 123, 7067–7073.
127. S. I. Klink, L. Grave, D. N. Reinhoudt, and F. C. J. M. van Veggel. A systematic study of the photophysical processes in polydentate triphenylene-functionalized Eu3+, Tb3+, Nd3+, Yb3+, and Er3+ complexes. J. Phys. Chem. A 2000, 104, 5457–5468.
128. W. D. Horrocks and M. Albin.In: S. J. Lippard,Ed., Progress in Inorganic Chemistry, John Wiley & Sons, 1984.
129. K. Binnemanns. Lanthanides and actinides in ionic liquids. Chem. Rev. 2007, 107, 2592–2614.
130. C. Piguet, J. C. G. Bünzli, B. Donnio, and D. Guillon. Thermotropic lanthanidomesogens. Chem. Commun. 2006, 3755–3768.
131. B. Donnio, D. Guillon, R. Deschenaux, and D. W. Bruce.In: J. A. McCleverty and T. J. Meyer, Eds., Comprehensive Coordination Chemistry II, vol. 7, Elsevier, Oxford, UK, 2003.
132. M. Yu, Y. J. Zhang, J. H. Shi, G. F. Liu, and H. J. Zhang. Holmium porphyrin compound liquid crystals: synthesis and properties. Solid State Sci. 2009, 11, 2016–2022.
133. D. Atilla, N. Kilinc, F. Yuksel, A. G. Gurek, Z. Z. Ozturk, and V. Ahsen. Synthesis, characterization, mesomorphic and electrical properties of tetrakis(alkylthio)-substituted lutetium(III) bisphthalocyanines. Synth. Met. 2009, 159, 13–21.
134. N. V. S. Rao, T. D. Choudhury, R. Deb, M. K. Paul, T. R. Rao, T. Francis, and I. I. Smalyukh. Fluorescent lanthanide complexes of Schiff base ligands possessing N-aryl moiety: influence of chain length on crossover (calamitic to discotic) phase behavior. Liq. Cryst. 2010, 37, 1393–1410.
135. L. A. Holt, R. J. Bushby, S. D. Evans, A. Burgess, and G. Seeley. A 106-fold enhancement in the conductivity of a discotic liquid crystal doped with only 1% (w/w) gold nanoparticles. J. Appl. Phys. 2008, 103, 063712.
136. S. Kumar and V. Lakshminarayanan. Inclusion of gold nanoparticles into a discotic liquid crystalline matrix Chem. Commun. 2004, 1600–1601.
137. D. Vijayaraghavan and S. Kumar. Self-assembled superlattices of gold nanoparticles in a discotic liquid crystal. Mol. Cryst. Liq. Cryst. 2009, 508, 101–114.
138. S. Kumar, S. K. Pal, P. S. Kumar, and V. Lakshminarayanan. Novel conducting nanocomposites: synthesis of triphenylene-covered gold nanoparticles and their insertion into a columnar matrix. Soft Matter 2007, 3, 896–900.
139. H. K. Bisoyi and S. Kumar. Carbon nanotubes in triphenylene and rufigallol-based room temperature monomeric and polymeric discotic liquid crystals. J. Mater. Chem. 2008, 18, 3032–3039.
140. S. H. Eichhorn, A. Adavelli, H. S. Li, and N. Fox. Alignment of discotic liquid crystals. Mol. Cryst. Liq. Cryst. 2003, 397, 47–58.
141. J. K. Vij, A. Kocot, and T. S. Perova. Order parameter, alignment and anchoring transition in discotic liquid crystals. Mol. Cryst. Liq. Cryst. 2003, 397, 231–244.
142. L. Schmidt-Mende, A. Fechtenkotter, K. Müllen, E. Moons, R. H. Friend, and J. D. MacKenzie. Self-organized discotic liquid crystals for high-efficiency organic photovoltaics. Science 2001, 293, 1119–1122.
143. O. Thiebaut, H. Bock, and E. Grelet. Face-on oriented bilayer of two discotic columnar liquid crystals for organic donor–acceptor heterojunction. J. Am. Chem. Soc. 2010, 132, 6886–6887.
144. N. Terasawa, H. Monobe, K. Kiyohara, and Y. Shimizu. Strong tendency towards homeotropic alignment in a hexagonal columnar mesophase of fluoroalkylated triphenylenes. Chem. Commun. 2003, 1678–1679.
145. W. Pisula, Z. Tomovic, B. El Hamaoui, M. D. Watson, T. Pakula, and K. Müllen. Control of the homeotropic order of discotic hexa-peri-hexabenzocoronenes. Adv. Funct. Mater. 2005, 15, 893–904.
146. R. I. Gearba, D. V. Anokhin, A. I. Bondar, W. Bras, M. Jahr, M. Lehmann, and D. A. Ivanov. Homeotropic alignment of columnar liquid crystals in open films by means of surface nanopatterning. Adv. Mater. 2007, 19, 815–820.
147. E. Charlet, E. Grelet, P. Brettes, H. Bock, H. Saadaoui, L. Cisse, P. Destruel, N. Gherardi, and I. Seguy. Ultrathin films of homeotropically aligned columnar liquid crystals on indium tin oxide electrodes. Appl. Phys. Lett. 2008, 92, 024107.
148. E. Pouzet, V. D. Cupere, C. Heintz, J. W. Andreasen, D. W. Breiby, M. M. Nielsen, P. Viville, R. Lazzaroni, G. Gbabode, and Y. H. Geerts. Homeotropic alignment of a discotic liquid crystal induced by a sacrificial layer. J. Phys. Chem. C 2009, 113, 14398–14406.
149. M. Steinhart, S. Zimmermann, P. Goring, A. K. Schaper, U. Gosele, C. Weder, and J. H. Wendorff. Liquid crystalline nanowires in porous alumina: geometric confinement versus influence of pore walls. Nano Lett. 2005, 5, 429–434.
150. D. Miyajima, K. Tashiro, F. Araoka, H. Takezoe, J. Kim, K. Kato, M. Takata, and T. Aida. Liquid crystalline corannulene responsive to electric field. J. Am. Chem. Soc. 2009, 131, 44–45.
151. H. Monobe, K. Awazu, and Y. Shimizu. Alignment control of a columnar liquid crystal for a uniformly homeotropic domain using circularly polarized infrared irradiation. Adv. Mater. 2006, 18, 607–610.
152. H. Monobe, H. Hori, Y. Shimizu, and K. Awazu. Alignment control of columnar liquid crystals for uniformly homeotropic domain with circularly polarized infrared irradiation. Mol. Cryst. Liq. Cryst. 2007, 475, 13–22.
153. S. Zimmermann, J. H. Wendorff, and C. Weder. Uniaxial orientation of columnar discotic liquid crystals. Chem. Mater. 2002, 14, 2218–2223.
154. A. M. van de Craats, N. Stutzmann, O. Bunk, M. M. Nielsen, M. Watson, K. Müllen, H. D. Chanzy, H. Sirringhaus, and R. H. Friend. Meso-epitaxial solution-growth of self-organizing discotic liquid-crystalline semiconductors. Adv. Mater. 2003, 15, 495–499.
155. W. Pisula, A. Menon, M. Stepputat, I. Lieberwirth, U. Kolb, A. Tracz, H. Sirringhaus, T. Pakula, and K. Müllen. A zone-casting technique for device fabrication of field-effect transistors based on discotic hexa-peri-hexabenzocoronene. Adv. Mater. 2005, 17, 684–689.
156. W. Pisula, M. Kastler, D. Wasserfallen, T. Pakula, and K. Müllen. Exceptionally long-range self-assembly of hexa-peri-hexabenzocoronene with dove-tailed alkyl substituents. J. Am. Chem. Soc. 2004, 126, 8074–8075.
157. T. Bjornholm, T. Hassenkam, and N. Reitzel. Supramolecular organization of highly conducting organic thin films by the Langmuir–Blodgett technique. J. Mater. Chem. 1999, 9, 1975–1990.
158. O. Y. Mindyuk and P. A. Heiney. Structural studies of Langmuir films of disc-shaped molecules. Adv. Mater. 1999, 11, 341–344.
159. P. Smolenyak, R. Peterson, K. Nebesny, M. Torker, D. F. O'Brien, and N. R. Armstrong. Highly ordered thin films of octasubstituted phthalocyanines. J. Am. Chem. Soc. 1999, 121, 8628–8636.
160. N. Reitzel, T. Hassenkam, K. Balashev, T. R. Jensen, P. B. Howes, K. Kjaer, A. Fechtenkotter, N. Tchebotareva, S. Ito, and K. Müllen. Langmuir and Langmuir–Blodgett films of amphiphilic hexa-peri-hexabenzocoronene: new phase transitions and electronic properties controlled by pressure. Chem. Eur. J. 2001, 7, 4894–4901.
161. H. J. Räder, A. Rouhanipour, A. M. Talarico, V. Palermo, P. Samorì, and K. Müllen. Processing of giant graphene molecules by soft-landing mass spectrometry. Nat. Mater. 2006, 5, 276–280.
162. I. O. Shklyarevskiy, P. Jonkheijm, N. Stutzmann, D. Wasserberg, H. J. Wondergem, P. C. M. Christianen, A. P. H. J. Schenning, D. M. de Leeuw, Z. Tomovic, J. Wu, K. Müllen, and J. C. Maan. High anisotropy of the field-effect transistor mobility in magnetically aligned discotic liquid-crystalline semiconductors. J. Am. Chem. Soc. 2005, 127, 16233–16237.
163. A. Calo, P. Stoliar, M. Cavallini, S. Sergeyev, Y. H. Geerts, and F. Biscarini. Monolayer control of discotic liquid crystal by electromigration of dewetted layers in thin film devices, J. Am. Chem. Soc. 2008, 130, 11953–11958.
164. K. Kawata. Orientation control and fixation of discotic liquid crystal. Chem. Rec. 2002, 2, 59–80.
165. T. L. Benanti and D. Venkataraman. Organic solar cells: an overview focusing on active layer morphology. Photosynth. Res. 2006, 87, 73–81.
166. B. A. Gregg, M. A. Fox, and A. J. Bard. Photovoltaic effect in symmetrical cells of a liquid crystal porphyrin. J. Phys. Chem. 1990, 94, 1586–1598.
167. K. Petritsch, R. H. Friend, A. Lux, G. Rozenberg, S. C. Moratti, and A. B. Holmes. Liquid crystalline phthalocyanines in organic solar cells. Synth. Met. 1999, 102, 1776–1777.
168. T. Christ, B. Glusen, A. Greiner, A. Kellner, R. Sander, V. Stumpflen, V. Tsukruk, and J. H. Wendorff. Columnar discotics for light emitting diodes. Adv. Mater. 1997, 9, 48–51.
169. I. H. Stapff, V. Stümpflen, J. H. Wendorff, D. B. Spohn, and D. Möbius. Multi-layer light emitting diodes based on columnar discotics, Liq. Cryst. 1997, 23, 613–617.
170. A. Bacher, I. Bleyl, C. H. Erdelen, D. Haarer, W. Paulus, and H. W. Schmidt. Low molecular weight and polymeric triphenylenes as hole transport materials in organic two-layer LEDs. Adv. Mater. 1997, 9, 1031–1035.
171. I. Seguy, P. Jolinat, P. Destruel, J. Farenc, R. Mamy, H. Bock, J. Ip, and T. P. Nguyen. Red organic light emitting device made from triphenylene hexaester and perylene tetraester. J. Appl. Phys. 2001, 89, 5442–5448.
172. T. Hassheider, S. A. Benning, H. S. Kitzerow, M. F. Achard, and H. Bock. Color-tuned electroluminescence from columnar liquid crystalline alkyl arenecarboxylates. Angew. Chem. Int. Ed. 2001, 40, 2060–2063.
173. H. Sirringhaus. Device physics of solution-processed organic field-effect transistors. Adv. Mater. 2005, 17, 2411–2425.
174. Y. Shimizu, K. Oikawa, K. Nakayama, and D. Guillon. Mesophase semiconductors in field effect transistors. J. Mater. Chem. 2007, 17, 4223–4229.
175. S. Cherian, C. Donley, D. Mathine, L. LaRussa, W. Xia, and N. Armstrong. Effects of field dependent mobility and contact barriers on liquid crystalline phthalocyanine organic transistors. J. Appl. Phys. 2004, 96, 5638–5643.
176. S. Tatemichi, M. Ichikawa, T. Koyama, and Y. Taniguchi. High mobility n-type thinfilm transistors based on N, N-ditridecyl perylene diimide with thermal treatments. Appl. Phys. Lett. 2006, 89, 112108.
177. R. Schmidt, J. H. Oh, Y. S. Sun, M. Deppisch, A. M. Krause, K. Radacki, H. Braunschweig, M. Konemann, P. Erk, Z. Bao, and F. Wurthner. High-performance air-stable n-channel organic thin film transistors based on halogenated perylene bisimide semiconductors. J. Am. Chem. Soc. 2009. 131, 6215–6228.
178. Q. Li,Ed., Self-organized Organic Semiconductors: From Materials to Device Applications, John Wiley & Sons, New Jersey, 2011.