Chapter 13
Fact or Fiction: Cybotactic Groups in the Nematic Phase of Bent Core Mesogens
13.1 Introduction
A defect-free crystalline solid possesses long-range bond-orientational and positional- order and, therefore, has low symmetry. Lattices of crystalline solids are invariant under symmetry operations corresponding to a specific space group and the basis of the crystal [1]. Isotropic liquids, on the other hand, exhibit positional–order correlations that extend over a very short distance, typically only up to few nearest neighbors. As a consequence, the isotropic (I) liquid is invariant under any (translational, rotational, or other) symmetry operation (Figure 13.1a). Some materials with non-spherical building blocks (e.g., rods, discs, parallelepipeds, and bent rods) exhibit a number of intermediate phases between the crystalline solid and isotropic liquid. These are collectively known as liquid crystals (LCs). The anisotropic shape of their building blocks effectively leads to anisotropic interaction potentials and to the development of long-range orientational order. Depending on specific molecular organization and the nature of interactions, a variety of LC phases with different (short or long) range of bond-orientational or positional order along different spatial directions have been predicted and observed [2].
Figure 13.1 Molecular organization in the (a) isotropic and (b) nematic phases of rod like molecules.
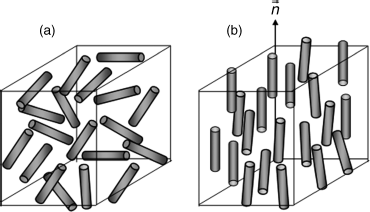
Among the various LC phases, the simplest is the nematic (N) phase in which the symmetry axis of the constituent entities, for example, long axes of cylindrical molecules, are on average aligned along a common direction, known as the director (), as shown in Figure 13.1b. The measure of orientational order, that is, the degree to which molecules are parallel to each other, is given by the nematic order parameter S = ½ < 3 cos2 θ − 1>, where θ is the average angle that individual molecules make with
and the brackets represent ensemble average. The N phase does not possess any translational order and its existence requires anisotropic interactions between anisotropic objects. This is generally achieved by designing molecules with a rigid core (to provide anisotropic interaction) and flexible end-groups (to render the interactions weak enough to prevent crystallization). Based on these criteria, a number of LCs have been designed, synthesized, and are widely used in commercial applications. Most of the molecules that form the N phase are cylindrically symmetric and the resulting N phase possesses uniaxial symmetry. In this chapter, we describe properties of the uniaxial N phase followed by the recent advances in understanding the structural properties of the biaxial nematic phases of bent-core molecules.
13.2 Nematic Phase of Rod-Like Molecules
When a LC material is cooled from the I phase toward the N–I transition temperature (TNI), small nematic volumes begin to spontaneously form and disappear as a consequence of nematic thermal fluctuations in the isotropic phase. In these correlated regions the nematic order parameter (OP), S, is non-zero and a fraction of the incident light energy is scattered due to miniscule turbidity in the proximity of the N phase. The orientation of local director of different clusters is uncorrelated and the cluster sizes are small. Within these clusters, the molecules exhibit local short-range orientational correlation that may extend up to 20–50 molecular dimensions [3]. Therefore, the isotropic phase optically appears dark between crossed polarizers. Typical time scale of the nematic OP fluctuations in the isotropic phase is in the range of micro- to milli-seconds [4].
At TNI, the system enters the N phase via a weakly first order phase transition. The N phase is characterized with three (Bent, Splay, and Twist) deformations with corresponding elastic constants. At the temperatures of interest, the energy needed to spontaneously affect a long wavelength deformation can be lower than or comparable to ½KBT. Consequently, the local director (r) fluctuates with a relaxation time that depends on the values of relevant elastic and viscosity coefficients. In a bulk nematic LC, and in the absence of external boundary conditions, these domains are randomly distributed. As a consequence, measurements of any macroscopic physical parameters, such as refractive index and dielectric constant, in an unaligned nematic LC yield an average contribution from these randomly oriented domains. Therefore, a uniformly aligned mono-domain, needed for fundamental studies or applications that exploit anisotropic physical properties of NLC is generally achieved by subjecting these microscopic domains to external fields, such as surface anchoring, magnetic, or electric fields.
As the temperature of the N phase is lowered toward an underlying smectic phase, the effect of the underlying phases starts to emerge in the vicinity of the transition temperature in the form of small correlated volumes having the properties and order belonging to the more ordered smectic phase [5]. Depending on the underlying phase, these local correlations can be short-range SmA or SmC like. These pre-transitional correlated volumes once were believed to be characteristics of a new thermodynamically stable phase [6]. The SmA/SmC fluctuations, with the molecules arranged perpendicular/oblique to smectic layers, are referred to as SmA/SmC cybotactic groups and skew–cybotactic groups, respectively. Molecular organization in these cybotactic groups is shown in Figure 13.2. The nature of intermolecular interactions and distance from the transition temperature determine the size and time scale of pre-transitional smectic fluctuations in the nematic matrix.
Figure 13.2 Cybotactic clusters in a nematic (N) matrix with (a) SmA-type and (b) SmC-type fluctuations. The LC molecules within the fluctuating smectic cluster are aligned parallel to the director, adapted from Ref. [5].
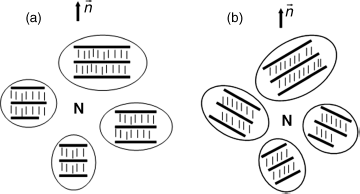
In most cases, the smectic cybotactic groups are present within a few degrees from the transition temperature [7] whereas in other systems they may persist over almost the entire nematic range of as much as 100°C [8]. In both cases, these smectic pre-transitional fluctuations in the N phase grow in size as the transition to lower phase is approached. As a consequence, physical parameters related to their effective size also show strong temperature dependence. For example, if the underlying phase is the SmA phase, both longitudinal and transverse correlation lengths (i.e., parallel and perpendicular to ) diverge with a power law at the transition [9], as depicted in Figure 13.3a. The evolution of SmC type (i.e., skew) cybotactic groups is reflected in (a) the divergence of the two correlation lengths (Figure 13.3b), (b) an increase in tilt angle, and (c) a decrease in layer spacing with decreasing temperature [10]. As extensive X-ray results [9, 11, 12] accumulated over the past three decades show, the area and the number of layers in correlated volumes increase with decreasing temperature. Since the first use of the term “cybotactic groups” by de Vries [6] and its adoption by McMillan [7], it has been synonymous with pretransitional fluctuations. There was little use of this term over the past 25 years. However, it has recently been used to describe the X-ray diffraction patterns from the thermotropic biaxial N phase in bent-core mesogens. In this chapter, we examine recent uses of the term cybotactic groups against the above listed expectations and the validity of their reported sightings.
Figure 13.3 Variation of longitudinal correlation length with temperature in the N phase of LCs (a) 8OCB and (b) 6OO8 possessing underlying SmA and SmC phases, respectively. The correlation length in the nematic phase grows as the underlying SmA or SmC phase is approached. The correlation lengths for 6OO8 were replotted from the values in Ref. [35].
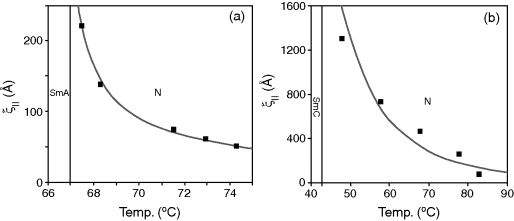
13.3 X-Ray Scattering
X-ray diffraction (XRD) is an ideal non-destructive tool for extracting the static and dynamical structural information about a system. XRD has been used to identify phases [2], quantitatively investigate critical phenomena [5, 11], and to obtain the structural information of solid and liquids [13]. For a system of N molecules, the total scattered intensity at any point in the -space is given by the average over all molecules and their relative orientations [11],
where, is the Fourier transform of the electron density
of ith atom in a molecule, < > represents ensemble average, and
is the X-ray momentum transfer vector. In general, the scattered intensity is expressed as a product of two terms: the form factor
that depends primarily on the molecular structure and conformation, and the structure factor
that includes both spatial and orientational correlations:
The structural factor depends on the relative positions and orientation of two neighboring anisotropic molecules and is given by [9],
In a system with infinitely narrow instrumental resolution function, the observed intensity in is given by
.
This formulism with appropriate approximations for the form and structure factors has been used to understand the structure of different phases. In the liquid phase of isotropic molecules, the correlations are mostly limited only to the nearest neighbors and can be approximated by the Fourier transform of the pair correlation function. Therefore, the diffraction pattern exhibits a diffuse ring in the reciprocal space that corresponds to the length scale associated with average nearest neighbor separation [13]. In the I phase of anisotropic (e.g., rod like) molecules, there are two length scales (length and the width) associated with nearest neighbor correlations. Therefore, two diffused rings corresponding to the two length scales are observed. The ring at the small (large) angle corresponds to the length (width) of the molecules. For a uniformly aligned (i.e., monodomain) N phase, the diffraction pattern reflects its symmetry. Consequently, the two diffuse rings in the isotropic phase condense into two pairs of diffuse crescents, one at small angle along
and the other along the orthogonal direction (Figure 13.4).
Figure 13.4 Two dimensional X-ray diffraction pattern from (a) isotropic and (b) nematic phases of 4-cyano-4′-pentylbiphenyl (5CB) and (c) cybotactic smectic C phase of nematic phase of bis-(4′-n-octyloxybenzal)-2-chlor1o,4-phenylenediamine. Vector represents the direction of external magnetic field while the white line in (c) denotes the direction of the capillary axis. Source: Reprinted with permission from de Vries [8], Copyright 1970, Gordon Breach Science Publishers. (See the color version of this figure in Color Plates section.)
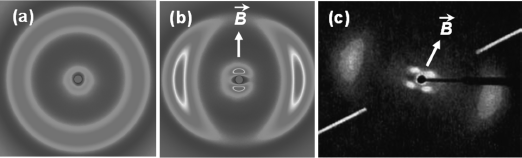
In the case of SmA cybotactic groups, the diffraction pattern is very similar to that of the ordinary N phase except that the inner diffuse peaks are sharper owing to stronger scattering from enhanced pre-transitional fluctuations. The “correlated volume” (or, cybotactic group size) diverges as temperature approaches the transition. Scattered intensity in these systems has been modeled using a Lorentzian-like structure factor combined with a slowly decaying function of q around q = 0. The form factor has been analytically calculated for the rod-like molecules to appropriately describe the observed intensity distribution [14]. The SmC fluctuations in the N phase give rise to four diffuse small angle peaks as a result of the degeneracy of smectic layer orientation with respect to which is along the field direction (Figure 13.2b). Experiments performed in the N phase with or without an underlying SmC phase have established that the correlation lengths in these systems increase, the apparent tilt angle increases, and the layer spacing, d, decreases as the temperature is lowered [7–12].
13.4 Nematic Phase of Bent Core Mesogens
Since the pioneering work of Niori et al. [15], it has become clear that the LC phases exhibited by bent-core mesogens possess properties not observed in calamitic mesogens. They have emerged as a new sub-field of LC science [16]. In the I phase, the bent core molecules are randomly distributed while in N phase, their molecular biaxiality leads to two different possibilities. When the long axes of molecules are aligned parallel to the primary director , and the planes of the molecules are randomly distributed around
, one obtains the well-known uniaxial N phase (Figure 13.5a). On the other hand, a biaxial N phase forms when the planes of the molecules are aligned parallel to the secondary director
(Figure 13.5b). XRD pattern from the I phase of bent-core LC consists of two diffuse rings corresponding to the effective length and width of molecules (Figure 13.6a). The XRD pattern from the nematic phase of these materials, however, depends on the ordering of the directors
and
(see below).
Figure 13.5 Molecular organization in (a) uniaxial and (b) biaxial nematic phase of bent-core molecules.
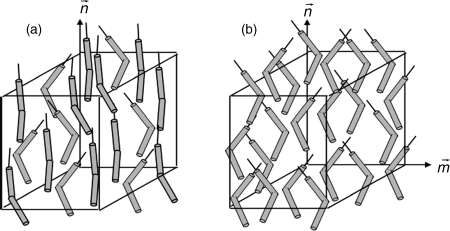
Figure 13.6 The 2D XRD from (a) isotropic and (b) the nematic phases of bent-core molecules. The diffraction at small angle in the N phase depends on the alignment of secondary director.
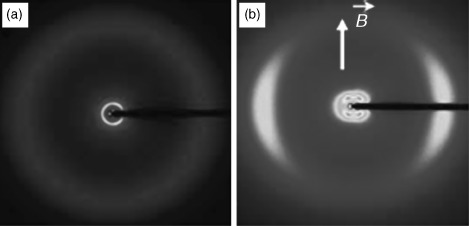
Among various bent-core molecules synthesized over the past decade, mesogens with rigid oxadiazole core exhibit a stable nematic phase [17]. The first attempt to investigate their structural properties in the nematic phase using XRD was carried out on three compounds (Figure 13.7) [18, 19]. An aligned nematic phase of these materials exhibited four small angle diffuse peaks very similar to that of SmC type cybotactic groups. However, the measured values of correlation lengths were comparable to molecular dimensions and independent of temperature over the entire N phase as has been independently confirmed by several research groups [20, 21]. Based on the very weak thermal dependence of correlation lengths, the possibility of pronounced pretransitional SmC fluctuations, i.e., the existence of large cybotactic groups can be categorically ruled out. Conventional approximations used in the analysis of X-ray diffraction patterns were not sufficient to fully describe the LC phases formed by these molecules. By sandwiching a thin film of LC between two rubbed polyimide-coated substrates and applying an external electric filed, the structure of the nematic phase in the two orthogonal directions in the plane perpendicular to , was also investigated. Two-dimensional XRD from the surface-aligned sample exhibited distinct patterns in the two orthogonal directions as shown in Figure 13.8 [18, 19].
Figure 13.7 Molecular structures of oxadiazole-based compounds exhibiting four diffuse XRD peaks at small angle.
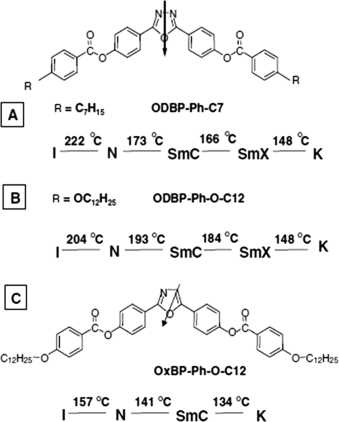
Figure 13.8 Two-dimensional XRD pattern from the nematic phase of (a) compound A at 195°C inside a capillary tube in the presence of magnetic field , (b) compound B between two beryllium plates coated with polyimide film and rubbed along
, and (c) sample in (b) in the presence of external electric field perpendicular to the substrates. Panels (b) and (c) show only the small angle diffraction for clarity.
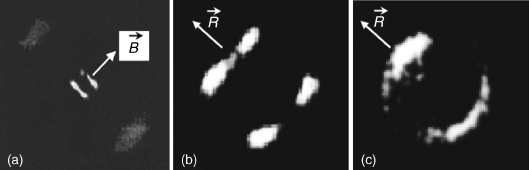
To describe these XRD patterns, a simple model of the nematic phase was developed. Bent-core molecules were approximated by two rigid cylinders with a fixed angle between them, a widely accepted approximation [22, 23]. Both, the form and the structure factors were used to calculate the scattering intensity for an aligned mono-domain nematic phase [14]. The calculated X-ray intensity distribution exhibited distinct patterns in the two orthogonal directions in the plane perpendicular to , in qualitative agreement with the experimental observation. This led to the discovery of theoretically predicted biaxial nematic phase in a low-molar mass oxadiazole-based bent core thermotropic LC [18, 19, 24, 25]. It should be noted that the claim of biaxial nematic phase was based on the observation of two distinct diffraction patterns in two orthogonal planes (Figure 13.8) and NOT just on mere existence of four diffuse peaks as has been erroneously stated [27] by some researchers.
To incorporate orientational fluctuations of and
, Mayer-Saupe type temperature dependent distribution function was introduced. Based on the parameters (e.g., the temperature and the interaction potential) of the distribution function, the model can predict isotropic and both uniaxial and biaxial nematic phases. The model was used to interpret the XRD pattern observed in the N phase of azo-based bent core LCs and to predict the presence of uniaxial and biaxial nematic phases [14, 26]. According to this model, origin of the four diffuse peaks in XRD pattern is the geometrical structure (i.e., the form factor) of the bent-core molecule and not the presence of cybotactic clusters. The model predicts that the XRD pattern from the N phase of mesogens that maintain the bent structure in the statistical ensemble, consists of four diffuse peaks independently of the underlying phase. The uniaxial N phase has identical diffraction patterns while the biaxial nematic phase must exhibit distinct patterns in the two orthogonal directions perpendicular to
.
Since the discovery of the biaxial N phase in oxadiazole-based bent-core LCs, different experimental techniques have been used to investigate these and other bent-core materials. Southern et al. [20] have studied, using XRD and polarized Raman scattering [PRS], biaxial order in oxadiazole-based bent core LC C5–Ph–OC12 with asymmetric alkyl chains (Figure 13.9). XRD from the nematic phase of these molecules exhibited four diffused peaks similar to the symmetric compounds [18]. While no attempt was made to align the planes of the molecules with a second field to extract the information on the biaxiality using XRD, the PRS measurements supported the existence of both uniaxial and biaxial nematic phases.
Figure 13.9 Molecular structure of compound C5-Ph-OC12 and the transition temperatures. Source: Reprinted with permission from Southern et al. [20]. Copyright 2008 EPLA.
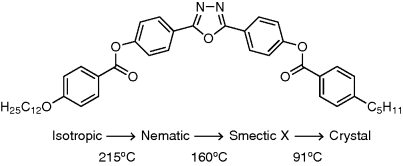
One of the most important results of this study was the temperature independence of the end-to-end (or, longitudinal) correlation length that was found to be comparable to the molecular dimension over the entire nematic range of more than 50°C (Figure 13.10). Also, angular separation between two XRD peaks slowly increased and saturated at ~15°C below TNI. These results, clearly demonstrate that the four diffuse peaks are not the result of SmC fluctuations but must arise from the changes in the bend angle of the core.
Figure 13.10 Temperature dependence of correlation length of C5-ODBP-ph-OC12. Source: Reprinted with permission from Southern et al. [20]. Copyright 2008 EPLA.
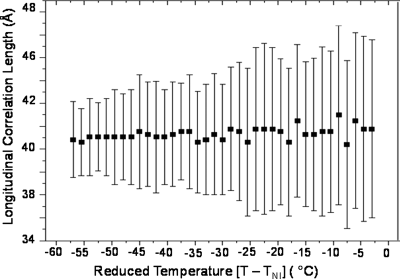
A group in Japan [16] conducted XRD study of a thiazole-based bent core LC with a 13°C wide N phase and an underlying SmC phase (Figure 13.11). Four diffuse peaks at small angles characterize the XRD patterns of this phase. They reported that the coherence length increased at lower temperatures suggesting the presence of cybotactic groups.
Figure 13.11 Molecular structure and X-ray diffraction patterns from molecules with long alkyl chains. Source: Reprinted with permission from Takazoe and Takanishi [16]. Copyright 2006, Japan Society of Applied Physics.
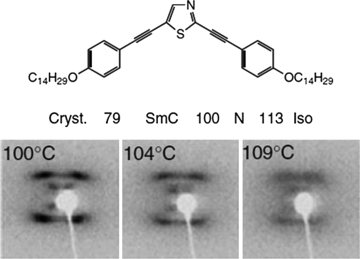
Vaupoti et al. [27], investigated bent-core LCs based on 3,4-benzothiophene (BTP) and 1,3 dimethoxybenzene (DMB) (compounds 1 and 2 in Figure 13.12). Two-dimensional XRD patterns from the LC phase of the BTP molecule having asymmetric arms exhibited four diffused peaks at small angle in the N phase above the SmC phase. The mesogen with hexyl group attached to the central DMB core is the first known bent-core system to exhibit a stable N phase above the SmA phase. XRD patterns of the DMB mesogen consist of two diffuse peaks at small angle. Similarly, a mixture of two bent-core molecules (compounds 3 and 4 in Figure 13.12), exhibiting the SmA and SmC phase, gave a stable N phase with underlying SmA phase and had only two small angle diffuse peaks. Based on these observations, the authors concluded that the four diffuse peaks at the small angle were due to pre-transitional SmC fluctuations (or, the skewed cybotactic groups) and not due to the architecture of bent-core molecules. The presence of an underlying SmC phase was considered a prerequisite for the formation of skew cybotactic groups. We believe this requirement to be ad hoc and can be true only for second order transitions. But the N to SmC phase transition is almost always found to be first order. A nematic phase may appear to be headed for a second order transition to the SmC phase at lower temperatures, but other more ordered phases can intervene and preempt the expected transition via a first order transition. In those systems, the SmC phase may not be observable. Thus, as the temperature is lowered and depending on the nature of molecules and intrinsic interactions, the pretransitional fluctuations may be of a different nature than of the lower temperature phase if the transition is first order. Whether a mesogen would show SmA-like or SmC-like pretransitional fluctuations in the N phase can, to some extent, be inferred from the behavior of its homologs. In fact, in early seventies when de Vries introduced the term “cybotactic” to describe the pretransitional fluctuations, one of the homologous series of rod-like molecules exhibited skewed cybotactic groups while the underlying phase was a crystalline solid [8]. Other homologs of the same material actually showed SmC-like pretransitional phenomena in the N phase above the SmC phase. Recently, new bent core LCs with a stable N phase above the SmA phase exhibiting four diffuse peaks in XRD have been reported (see below).
Figure 13.12 Bent-core molecules used to establish that the four diffuse XRD peaks arise from SmC type fluctuations. Source: Reprinted with permission from Vaupoti et al. [27]. Copyright 2009, The American Physical Society.
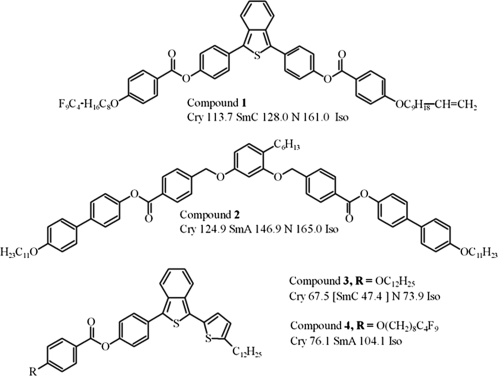
A random distribution of the cybotactic groups around the director was used in this study to model the average structure factor to represent the observed scattered X-ray intensity [27]. Although the temperature dependence of the correlation length or the tilt angle was not measured, the correlation lengths at a temperature few degrees above the smectic transition temperature was found to be five molecular lengths/widths along the directions longitudinal/transverse to . On the basis of limited reported information, it is not possible to conclude whether the changes in their diffraction patterns arose from thermal evolution of the structure factor (i.e., cybotactic groups) or from the changes in the molecular form factor arising from changes in molecular conformation or the bend-angle.
In another experiment [28], structural investigations of asymmetric 3,5-bis-{4-[4-(nonyloxy)benzoyloxy]phenyl}-1,2,4-oxadiazoles (9BPO) were conducted under the influence of surface anchoring and an applied electric field. This LC material exhibits the SmC phase below the N phase (Figure 13.13). The two-dimensional X-ray diffraction pattern from magnetic field aligned sample in the N phase reveals four diffuse peaks at small angle. The longitudinal and transverse correlation lengths increase with decreasing temperature as expected for skewed cybotactic group (Figure 13.14). Response to an applied electric field and the observed ferroelectric behavior both suggest that these molecules form SmC type polar cybotactic clusters that are randomly distributed in an unaligned N phase. Application of external electric field aligns these clusters along the field direction. Molecular dynamics simulations based on Gay-Berne dipolar model, where the bent-core molecule was approximated by three ellipsoids, supported the observed field-switching behavior. Although this explanation appears convincing at a first glance, it raises several serious questions.
Figure 13.13 Chemical structure and phase sequence of oxadiazole based compound (9BPO) showing ferroelectric nematic phase. Source: Reprinted with permission from Francesangeli et al. [28]. Copyright 2009 WILEY-VCH Verlag GmbH & Co. KGaA, Weinheim.
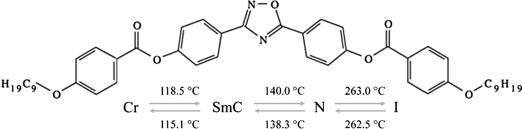
Figure 13.14 Correlations lengths of 9BPO at different magnetic field strengths as a function of temperature. Reprinted with permission from Francesangeli et al. [28], Copyright 2009 WILEY-VCH Verlag GmbH & Co. KGaA, Weinheim.
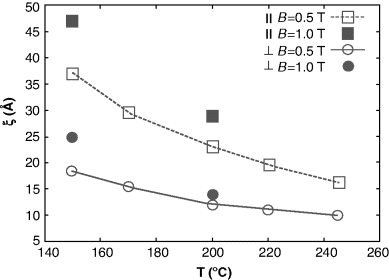
Firstly, the long dimension of these clusters (i.e., the longitudinal correlation length) is almost one molecular dimension under high magnetic field even close to the N – SmC transition! The transverse correlation length ranges from 2–4 molecular dimensions. If these polar cybotactic clusters do exist in this system, the correlated volumes is comprised of 4–16 molecules, a number very small for pretransitional fluctuations close to the N – SmC transition. Incidentally, if one assumes free rotation of the molecule about its long axis in the uniaxial N phase at 200°C, the transverse correlation length (~10 Å) turns out to be the average molecular width calculated using the 46 Å length and 132° bend angle [29]. Under this assumption, the transverse correlation length remains approximately 1–2 molecular lengths, thus contradicting the presence of cybotactic groups.
Second, when a thin film of LC is filled between SiO2 deposited glass substrates, the director aligns parallel to the deposition direction and four diffuse XRD peaks are observed [28]. However, in the presence of an electric field, molecular dipole moments (perpendicular to ) of the clusters rotate to become parallel to the electric field. It is inconceivable that
of these clusters previously aligned parallel to the rubbing direction become randomly oriented in the presence of the electric field! Perhaps a stronger field (surface or magnetic) that does not couple with macroscopic polarization of the clusters will produce an aligned monodomain of ferroelectric biaxial nematic phase to resolve this ambiguity.
A systematic temperature dependent small angle XRD from magnetically aligned N phase of the diester of 2,5-bis-(p-hydroxyphenyl)-1,3,4-oxadiazole (ODBP-Ph-O-C4H9) (Figure 13.15), which has no underlying SmC phase, has recently been performed [30]. The XRD pattern of this material consists of four diffuse peaks as reported by de Vries in a calamitic LCs [8] without an underlying SmC phase. This rules out the interpretation of the four diffuse XRD peaks as being indicative of the underlying pretransitional SmC cybotactic groups. XRD results from this material shows a “layer spacing” significantly lower than the actual molecular length (27.8 vs. 36 Å) leading to an estimated molecular tilt angle of 40.8°C at 210°C. Subsequently, a supramolecular structural model based on the SmC cybotactic clusters in a nematic host was proposed. X-ray scattering intensity profiles show very little peak sharpening with decreasing temperature. The calculated correlation lengths in two orthogonal directions are of the order of molecular dimensions and essentially independent of temperature over the entire nematic range (Figure 13.16). More importantly, the splitting of four small angle diffuse peaks is strongly temperature dependent. The origin of this change in splitting was attributed to the change in the tilt angle of the cybotactic groups. However, in the absence of any increase in SmC correlations (and thus the structure factor), the source of the splitting must be the changes in the form factor, very likely arising from changes in the bent-angle. Other investigations of such materials have shown a change in the bend angle as high as 27° [31, 32]. Again, if one assumes that molecules are free to rotate around their long axis as in the uniaxial N phase, the transverse correlation lengths corresponds to the diameter of the rotational volume of the molecule. Even with restricted rotation in cybotactic clusters, the number of molecules inside one correlated volume close to the transition remains ~10, inconsistent with the pretransitional cybotactic clusters which are known to diverge in size (Figure 13.3).
Figure 13.15 Structure of ODBP-ph-O-C4-H9, a homolog of ODBP-ph-OC12. Source: Reprinted with permission from Francesangeli and Samulski [30]. Copyright 2010, The Royal Society of Chemistry.

Figure 13.16 Measured correlation lengths in two orthogonal directions in ODBP-ph-O-C4H9. Reprinted with permission from Francesangeli and Samulski [30], Copyright 2010, The Royal Society of Chemistry.
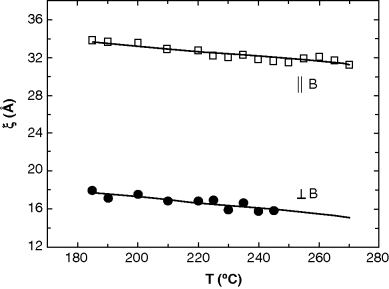
The N phase of ODBP-Ph-O-C4H9, between two substrates providing planar boundary conditions with provision to apply orthogonal electric and magnetic fields, has also been reported [30]. As observed earlier [18, 19] in the absence of external fields, the XRD shows four diffuse peaks [two of which are shown in Figure 13.17(a)]. When an external electric field is applied perpendicular to the cell, the diffraction pattern exhibits weak diffuse peaks approximately along the rubbing direction [Figure 13.17(b)]. With an in-plane magnetic field perpendicular to the electric field, the four small-angle diffuse peaks merge into two [Figure 13.17(c) shows one] with the maximum intensity along the magnetic field direction . These results suggest that
is aligned along
while
is oriented parallel to the electric field. These observations are qualitatively the same as earlier reported [18, 19] for the ODBP mesogens (Figure 13.8) to prove the nematic phase's biaxiality. These results, in particular, the correlation length remaining comparable to molecular dimensions over the entire nematic range and the change of four diffuse peaks into two upon application of two crossed fields strongly suggest that the origin of the four diffuse peaks in XRD patterns from these materials are not cybotactic clusters. Clearly, the four nematic peaks originate from the molecular structure of the mesogens per the model previously developed for ODBP [18, 19].
Figure 13.17 The 2D XRD patterns from nematic phase of ODBP-ph-O-C4H9 between two surfaces under different fields “r” represents the rubbing direction. (a) E = 0, B = 0: two peaks are evident from the two lobes seen in the outline of the reflection, (b) E = 4 V/μm, B = 0: the peaks nearly disappear as becomes parallel to
, (c) E = 4 V/μm, B = 1 T: lobes disappear as the two reflections become one and
orients || to
,
|| to
, and (d) E = 5V/μm, B = 1 T: higher electric field reorients
parallel to the electric field and the small angle peak disappears. Source: Reprinted with permission from Francesangeli and Samulski [30]. Copyright 2010, The Royal Society of Chemistry.
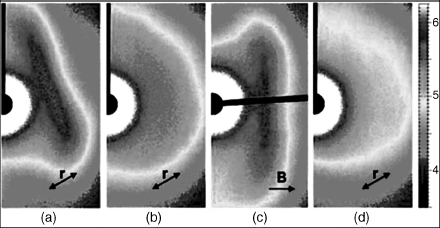
Structural investigations of ODBOP-ph-OC12 and ODBP-Ph-C7 also revealed [21] four diffuse peaks at small angle. Although the effect of crossed fields was not investigated, measured values of the correlation lengths confirmed that they remained comparable to molecular dimensions and were independent of temperatures. The tilt angle calculated from XRD shows a decrease of ~5° and 7° for ODBOP-ph-OC12 and ODBP-Ph-C7, respectively. The skewed cybotactic clusters were invoked to describe the observed four diffuse peaks. Meta-cybotactic structure, a more complex clustering than skewed cybotactic groups [33], was also suggested.
Based on results from different research groups on ODBP-based bent-core LCs, it can be concluded that (i) the nematic phase of these materials exhibits four diffuse peaks at small angle independently of the underlying phase, (ii) the separation between the peaks or the apparent tilt angle decreases with temperature, (iii) the “layer spacing” increases with temperature, and (iv) the positional order (or smectic) correlation lengths in these systems are of the order of molecular dimensions over the entire nematic temperature range. Under these conditions, thermal evolution of the tilt angle can only be explained by assuming some change in molecular bend angle and therefore the effective length of the molecules. These results provide strong evidence against the presence of the skewed cybotactic clusters as introduced by de Vries and McMillan, and subsequently adopted by de Gennes and others to describe the pretransitional fluctuations.
Keith et al. [34] have performed a systematic investigation of structural–property relationship in the N phase of a homologous series of bent-core molecules derived from 4-cyanoresorcinol with terminal alkyl chains of different lengths (Figure 13.18). Some of the homologs also have a smectic phase at lower temperatures. All members of the series exhibit four XRD diffuse peaks at small angles. The results also show that molecular organization in their N phase depends strongly on the length of the alkyl chains. For the two shortest (m = n = 2 and 4) homologs, the splitting between small-angle XRD peaks decreases with increasing temperature. In contrast, for all other homologs, the splitting increases and the layer spacing decreases with increasing temperature, a drastically different result from the ODBP-based bent core molecules. The layer spacing increases as a function of the number of alkyl chains but it is always smaller than the calculated molecular length (Figure 13.19(c)). Structural properties of the nematic phase of these materials reveal a transformation from a nematic phase having only the nearest neighbor correlations (for short chain molecules) as in ordinary nematics to large cybotactic clusters (for long chain molecules). LC materials with medium sized clusters (e.g., n = 9) have strong temperature dependent cluster sizes and therefore exhibit temperature dependent structural transformation. For shorter chain mesogens, the four diffuse peaks clearly arise from the molecular form factor (i.e., changes in the bend-angle) while for the longer chain LCs, the changes in the four diffuse peaks may originate from changes in tilt angle within the cybotactic clusters. It is expected that the bend-angle in all cases would change with temperature. However, clustering may overshadow it for larger molecules. For medium chain lengths, the changes in the four diffuse peaks can arise from changes in both the bend-angle (molecular geometry) and the molecular tilt angle within cybotactic clusters. Therefore, the organization of molecules in the N phase of bent core molecules is governed by detailed molecular structures, intermolecular interactions, geometrical factors, and clustering.
Figure 13.18 Molecular structure of cyanoresorcinol-based bent core molecules. The values of m and n was varied from 2 to 14. Source: Reprinted with permission from Keith et al. [34]. Copyright 2010, The Royal Society of Chemistry.
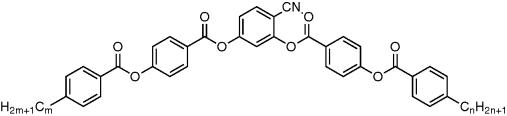
Figure 13.19 Temperature and chain length dependence of structural parameters of cyanoresorcinol based bent-core masogens. Δχ is the angular separation between diffuse peaks, d is the layer spacing and Lmol is molecular length. Source: Reprinted with permission from Keith et al. [34]. Copyright 2010, The Royal Society of Chemistry.
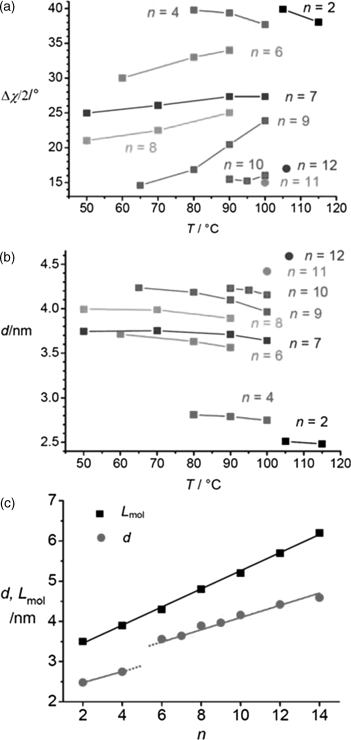
A different class of bent core molecules such as 4-chloro-1,3-phenylene bis-4-[40-(9-decenyloxy)benzoyloxy]benzoate (ClPbis10BB) and 4,6-dichloro-1,3-phenylene bis-4-[40(dodecyloxy)) biphenyl] carboxylate (DClPbis12BC) [35] is based on chlorophenylene at the center that defines the bend angle (Figure 13.20). These molecules are particularly attractive because they do not have any underlying smectic phase and, more importantly, they exhibit an unusually large flexoelectric effect [36] and flow viscosities [37]. XRD patterns of these compounds show two small angle arcs close to TNI that gradually transforms into four diffuse peaks in a manner similar to the other bent core systems. Quite interestingly, the length scales (i.e., layer spacing) are significantly smaller than the molecular lengths (32 Å vs. 45 Å). The observed XRD patterns are described on the basis of the existence of short-range SmC type correlations. Assuming that local director of these clusters follows Mayer-Saupe type distribution function, the experimentally observed diffraction patterns at small angle have been qualitatively reproduced. The longitudinal and transverse correlation lengths were found to be a few molecular dimensions and insensitive to temperature (Figure 13.21). For comparison, the correlation lengths for 6OO8, a molecule with structure similar to one of the arms of ClPbis10BB, is also plotted in Figure 13.21. Compound 6OO8 has the SmC phase below the N phase and is known to exhibit pretransitional fluctuations. The results clearly show that the correlation lengths (normalized with respect to the length of the molecules) are almost independent of temperatures for both bent core molecules while they are strongly temperature dependent for 6OO8. This clearly indicates that de Vries skewed cybotactic cluster model introduced to describe the pretransitional phenomena does not describe the four XRD diffuse peaks in this system. However, intermolecular correlations do exist in the N phase which extends up to several molecular dimensions in both directions. Supramolecular structures that involve a few molecules with smectic like correlations are most likely responsible for the observed XRD pattern in these systems.
Figure 13.20 Molecular structure and the transition temperatures of bent core molecules exhibiting unusual viscoelastic properties. 6OO8, a LC material exhibiting SmC phase below N phase, was studied for comparison. Source: Reprinted with permission from Hong et al. [35]. Copyright 2010, The Royal Society of Chemistry.
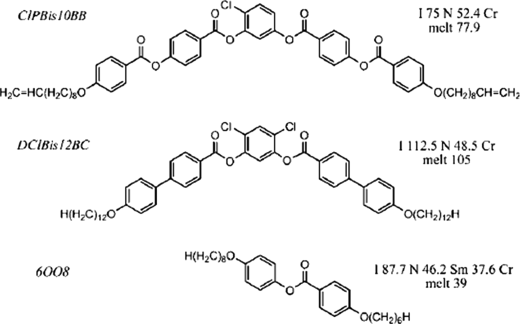
Figure 13.21 Temperature dependence of transverse and longitudinal correlation lengths (normalized with respective length of molecules) for ClPbis10BB, DClPbis12BC and 6OO8. The correlated lengths are plotted using the data from Table 1 in Ref. [35].
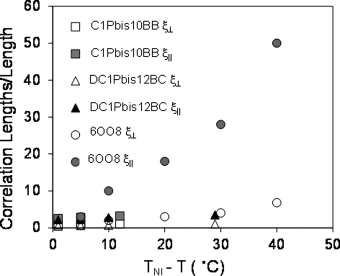
13.5 Summary
We have reviewed several reports on different forms of cybotactic clustering in the nematic phase of bent core molecules published since the discovery of the biaxial nematic phase [17, 18]. We have attempted to interpret results with the same criteria applied to all and commented on the validity of the interpretation of experimental results. It is made clear that the molecular organization/association in these systems is rather complex in the N phase. Depending on the specific molecular architecture, flexibility, and intermolecular interactions, the nematic phase of bent core molecules may behave like an ordinary nematic or as a nematic with self-organized nanoscopic clusters. While the XRD pattern in the first case can be explained by simple geometrical considerations, the interpretations of the second case requires the formation of supramolecular structures that might be responsible for unusual properties, such as ferroelectricity, giant flexoelectric effect, and anomalous viscoelastic coefficient. In either case, the correlation lengths appear to be independent of temperature for a number of bent core molecules. This suggests that the terminology “cybotactic groups” that has conventinoally been used to represent pretransitional phenomena, to describe statistical clustering of a few molecules which persist even into the isotropic phase appears to be misleading. This work was supported by Basic Energy Sciences, Office of Science, the US Department of Energy grant # DE-SC0001412. The authors thanks Dr. D. M. Agra-Kooijman for her sharing correlation length data.
1. C. Kittel. Introduction to Solid State Physics, 8th ed., John Wiley & Sons, 2005.
2. S. Kumar, Ed. Liquid Crystals: Experimental Study of Physical Properties and Phase Transitions, Cambridge University Press, 2001.
3. G. W. Stewart. X-ray diffraction intensity of the two liquid phases of para-azoxyanisol. J. Chem. Phys. 1936, 4, 231–236.
4. T. W. Stintson and J.D. Litster. Temperature dependence of normal modes in a nematic liquid crystals. Phys. Rev. Lett. 1970, 25, 503–506.
5. P. G. de Gennes. The Physics of Liquid Crystals, Oxford University Press, 1974.
6. A. de Vries. Evidence for the existence of more than one type of nematic phase. Mol. Cryst. Liq. Crys. 1970, 10, 31–37.
7. W. McMillan. Measurement of smectic-A-phase order-parameter fluctuations in the nematic pahse of p-n-Octyloxybenzylidene-p′-toluidine. Phys. Rev. A 1973, 7, 1673–1678.
8. A. de Vries. X-ray photographic studies of liquid crystals I: a cybotactic nematic phase. Mol. Cryst. Liq. Cryst. 1970, 10, 219–236.
9. A. Primak, M. Fisch, and S. Kumar. Critical behavior at the nematic-to-smectic-A transition in a strong magnetic field. Phys. Rev. Lett. 2002, 88, 035701–1-4.
10. A. de Vries. X-ray diffraction studies of structure of skewed cybotactic nematic pahse: A review of the literature. J. Mol. Liq. 1986, 31, 193–202.
11. J. Als-Nielsen, R. J. Birgeneau, M. Kaplan, J. D. Litster, and C. R. Safinya. High-resolution x-ray study of a second-order nematic-smectic-A phase transition. Phys. Rev. Lett. 1977, 39, 352–355.
12. J. Als-Nielsen, J. D. Litster, R. J. Birgeneau, M. Kaplan, C. R. Safinya, A. Lindegaard-Andersen, and S. Mathiesen. Observation of algebraic decay of positional order in a smectic liquid crystal. Phys. Rev. B 1980, 22, 312–320.
13. P. M. Chaikin and T. C. Lubensky. Principles of Condensed Matter Physics, Cambridge University Press, Cambridge, 1997.
14. B. R. Acharya, S. W. Kang, V. Prasad, and S. Kumar. Role of molecular structure on x-ray diffraction in uniaxial and biaxial phases of thermotropic liquid crystals. J. Phys. Chem. B 2009, 113, 3845–3852.
15. T. Niori, T. Sekine, J. Watanabe, T. Furukawa, H. Takezoe. Distinct ferroelectric smectic liquid crystals consisting of banana shaped achiral molecules. J. Mater. Chem. 1996, 6, 123–1233.
16. H. Takazoe and Y. Takanishi. Distinct ferroelectric smectic liquid crystals consisting of banana shaped achiral molecules. Jpn. J. Appl. Phys. 2006, 45, 597–625.
17. T. J. Dingemans and E. T. Samulski. Distinct ferroelectric smectic liquid crystals consisting of banana shaped achiral molecules. Liq. Cryst. 2000, 27, 131–136.
18. B. R. Acharya, A. Primak, T. J. Dingemans, E. T. Samulski, and S. Kumar. The elusive thermotropic biaxial nematic phase in rigid bent-core molecules. Pramana 2003, 61, 231–237.
19. B. R. Acharya, A. Primak, and S. Kumar. Biaxial nematic phase in bent-core thermotropic mesogens. Phys. Rev. Lett. 2004, 92, 145506-1-4.
20. C. D. Southern, P. D. Brimicomb, S. D. Siemianowski, S. Jaradat, N. Roberts, V. Gortz, J. W. Goodby, and H. F. Gleeson. Thermotropic biaxial nematic order parameters and phase transitions deduced by Raman scattering. EPL 2008, 82, 56001-1-6.
21. O. Francesangeli, F. Vita, C. Ferrero, T. Dingemans, and E. T. Samulski. Cybotaxis dominates the nematic phase of bent-core mesogens: a small-angle diffuse X-ray diffraction study. Soft Matter 2011, 7, 895–901.
22. D. R. Link, G. Natale, R. Shao, J. E. Maclennan, N. A. Clark, E. Korblova, and D. M. Walba. Spontaneous formation of macroscopic chiral domains in a fluid smectic phase of achiral molecules. Science 1997, 88, 1924–1927.
23. M. A. Bates. Influence of flexibility on the biaxial nematic phase of bent core liquid crystals: A Monte Carlo simulation study. Phys. Rev. E 2006, 74, 061702-1-11.
24. L. A. Madsen, T. J. Dingemans, M. Nakata, and E. T. Samulski. Thermotropic biaxial nematic liquid crystals. Phys. Rev. Lett. 2004, 92, 145505-1-4.
25. J. Palaez and M. R. Wilson. Atomistic simulations of a thermotropic biaxial liquid crystal. Phys. Rev. Lett. 2006, 97, 267801-1-4.
26. V. Prasad, S. W. Kang, K. A. Suresh, L. Joshi, Q. B. Wang, and S. Kumar. Thermotropic uniaxial and biaxial nematic and smectic phases in bent-core mesogens. J. Am. Chem. Soc. 2005, 127, 17224–17227.
27. N. Vaupoti, J. Szydlowska, M. Salamonczyk, A. Kovarova, J. Svoboda, M. Osipov, D. Pociecha, and E. Gorecka. Structure studies of the nematic phase formed by bent-core molecules. Phys. Rev. E 2009, 80, 030701(R)-1-4.
28. O. Francesangeli, V. Stanic, S. I. Torgova, A. Strigazzi, N. Scaramuzza, C. Ferrero, I. P. Dolbnya, T. M. Weiss, R. Berardi, L. Muccioli, S. Orlandi, and C. Zannoni. Ferroelectric response and induced biaxiality in the nematic phase of a bent-core mesogen. Adv. Funct. Mat. 2009, 19, 2592–2600.
29. S. I. Torgova, T. A. Geivandova, O. Francesangeli, and A. Strigazzi. Banana-shaped 1,2, 4-oxadiazoles. Pramana 2003, 61, 239–248.
30. O. Francesangeli and E. T. Samulski. Insights into the cybotactic nematic phase of bent-core molecules. Soft Matter 2010, 6, 2413.
31. R. Y. Dong and A Marini. Conformational study of a bent-core liquid lrystal: C-13 NMR and DFT computation approach. J. Phys. Chem. B 2009, 113, 14062–14072.
32. A. Eremin, H. Nadasi, G. Pelzl, S. Diele, H. Kresse, W. Weissflog, and S. Grande. Paraelectric–antiferroelectric transitions in the bent-core liquid-crystalline materials. Phys. Chem. Chem. Phys. 2004, 6, 1290–1298.
33. E. T. Samulski. Meta-cybotaxis and nematic biaxiality. Liq. Cryst. 2010, 37, 669–678.
34. C. Keith, A. Lehmann, U. Baumeister, M. Prehm, and C. Tschierske. Nematic phases of bent-core mesogens. Soft Matter 2010, 6, 1704–1721.
35. S. H. Hong, R. Verduzco, J. C. Williams, R. J. Twieg, E. DiMasi, R. Pindak, A. Jakli, J. T. Gleeson, and S. Sprunt. Short-range smectic order in bent-core nematic liquid crystals. Soft Matter 2010, 6, 4819–4827.
36. J. Harden, B. Mbanga, N. Éber, K. Fodor-Csorba, S. Sprunt, J. T. Gleeson, and A. Jákli. Giant flexoelectricity of bent-core nematic liquid crystals. Phys. Rev. Lett. 2006, 97, 157802-1-4.
37. S. Stojadinovic, A. Adorjan, and S. Sprunt. Giant flexoelectricity of bent-core nematic liquid crystals. Phys. Rev. E 2002, 66, 060701-1-4 (R).