Chapter 3
Magnetic Liquid Crystals
3.1 Introduction
Paramagnetic liquid crystals (PLCs) have been considered to become novel advanced soft materials that can combine the optical and electrical properties of conventional liquid crystals (LCs) with the magnetic and electronic properties of paramagnetic compounds [1–3]. The PLCs are classified into two categories: the majority were metal-containing LCs (metallomesogens) with permanent spins originating from transition (d-block) or lanthanide (f-block) metal ions in the mesogen core [3, 4], while only a few all-organic radical liquid-crystalline (LC) materials were prepared, most likely due to the difficulty in the molecular design and synthesis which must satisfy the molecular linearity or planarity necessary for the existence of LC phases (rod-like or disk-like molecules, respectively) as well as the radical stabilization [1, 5–7]. Moreover, endowing the PLCs with chirality is expected to result in the emergence of unconventional magneto-electric [8–10] or magneto-optical [11–13] properties, intriguing magnetic interactions and so on in the chiral LC state.
Thus, the research on chiral and achiral PLCs is being explored in the context of the following objectives or expectations:
where M is the magnetization, H is the applied magnetic field, E is the applied electric field, P is the polarization, χm is the magnetic susceptibility, χe is the electric susceptibility, and χme and are the magneto-electric susceptibilities.
For objective 1, the larger the magnetic anisotropy (Δχ), the weaker the critical magnetic field (Hc). This is because the Hc necessary for the alignment of LC molecule is inversely proportional to the square root of Δχ (Eq. (3.3)) [15].
where d represents the cell thickness and k is the elastic constant. For objective 2, there had been no precedent for a magnetically anisotropic chiral or achiral all-organic radical LC compound with a geometrically fixed spin unit in the core portion until the first PROXYL type of chiral LC compounds 1 were prepared by the present authors [16] (Figure 3.1). For objective 3, there was no report on the observation of appreciable magnetic interactions in PLCs until our recent finding of inhomogeneous magnetic interactions generated in the LC phases of compounds 1 under weak magnetic fields [17, 18]. For objective 4, such FLCs, if available, would need to exhibit both magnetic and electric ordering in the LC state [8–10]. As the typical examples of objective 5, Nakatsuji et al. observed the change in magnetic behavior between the original crystalline phase and the supercooled solid phase formed by cooling from the isotropic phase with respect to an all-organic PLC compound (see Section 3.5.1) [19], while Veciana et al. prepared a single-molecule magnet (SMM) with two different relaxation processes by controlling the cooling rate from the LC phase of a double-decker terbium complex (see Figure 3.11) [20]. Objective 6 has become one of the current topics in the research field of metallomesogens (see Sections 3.4.1.3 and 3.4.2.2). In the case of objective 7, Rikken et al. observed MChD in luminescence from an optically active paramagnetic Eu(III) complex in solution [11, 12] and in the enantiopure single crystal of a molecule-based ferromagnet composed of [Mn(II)Cr(III)(C2O42−)3]− and [N(CH3)(n-C3H7)2(s-C4H9)]+ [13]. However, MChD has not been observed yet in a chiral LC phase with magnetic ordering.
Figure 3.1 PROXYL type of LC compounds 1–5.
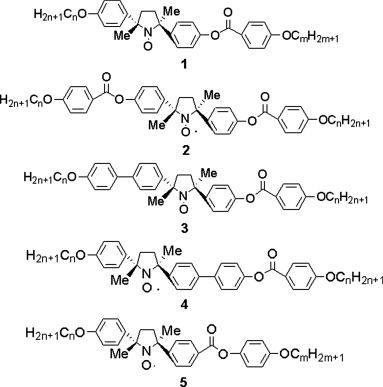
In this chapter, we first focus on the magnetic susceptibility anisotropy (or simply, magnetic anisotropy) of LCs that are relevant to the alignment of both diamagnetic and paramagnetic LC molecules by magnetic fields. Then magnetic properties of known paramagnetic metallomesogens and the first-generation of all-organic radical LCs are surveyed. In Section 3.5.3, our works on the second-generation of chiral all-organic radical LCs carried out in relation to the above objectives (2 and 3) are described briefly.
3.2 Magnetic Anisotropy (Δχ) of LCs
Similar to the molecular dielectric anisotropy (Δε), Δχ is obtained by subtracting the magnetic susceptibility component (χ⊥) perpendicular to the molecular long axis from the component (χ) parallel to the same axis (Figure 3.2 and Eq. (3.4)). Furthermore, Δχ comprises a paramagnetic component (Δχpara) (Eq. (3.5)) and a diamagnetic component (Δχdia) (Eq. (3.6)). Although χpara and χdia are always positive and negative, respectively, Δχpara and Δχdia become positive or negative, depending on the magnitude of the respective χ⊥ and χ
values. Therefore, the overall molecular magnetic anisotropy (Δχoverall) is the sum of Δχpara and Δχdia (Eq.(3.7)) [2, 3]. If Δχoverall is positive (or negative), the molecular long axis or the director becomes parallel (or perpendicular) to the applied magnetic field (H0), when the applied field is larger than Hc (Figure 3.2). Such is a driving force for molecular alignment by magnetic fields.
Figure 3.2 Magnetic anisotropy (Δχ) of LCs.
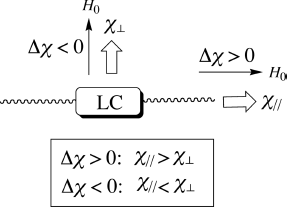
3.3 Diamagnetic LCs
Diamagnetism resides in all atoms. Particularly aromatic rings show a strong diamagnetic effect in an applied magnetic field. Therefore, the diamagnetic rod-like LC molecules orient themselves such that the axis with the most negative χdia is perpendicular to the magnetic field. Since χdia ⊥
is usually larger than
χdia
with respect to organic LC molecules, the Δχdia becomes positive and the molecules orient with the director parallel to the magnetic field (Figure 3.2). For organic LCs, the magnitude of the Δχdia, which is produced by two diamagnetic phenyl groups, is approximately +50 × 10−6 emu mol−1 [21]. Accordingly, a relatively strong magnetic field (>0.2 T) is necessary to align diamagnetic LCs, depending on the type of LC phases [22].
3.4 Paramagnetic Metallomesogens
Transition metal-containing metallomesogens which have the ligand-coordinated metal-complex structures with a number of long alkyl chains show nematic, smectic, or columnar mesophases [23], while lanthanide-containing LCs usually exhibit smectic or columnar mesophases with high viscosity due to their high and variable coordination numbers [24–26].
3.4.1 d-Block Metal Complexes
3.4.1.1 Magnetic Anisotropy
A number of paramagnetic rod-like metallomesogens containing 3d transition metal ions, such as VO(IV) (3d1), Fe(III) (3d5), Cu(II) (3d9) and Ni(II) (3d8), were synthesized and their magnetic properties were fully characterized (e.g., Figure 3.3) [2, 27–31]. Since the magnitude of the Δχpara arising from the 3d orbital is as small as 20–90 × 10−6 emu mol−1 and comparable to that of the Δχdia of organic ligands, the Δχpara and the Δχdia work cooperatively or competitively to determine the direction of the molecular alignment by magnetic fields [22, 32].
Figure 3.3 Transition metal-containing metallomesogens and their magnetic anisotropy.
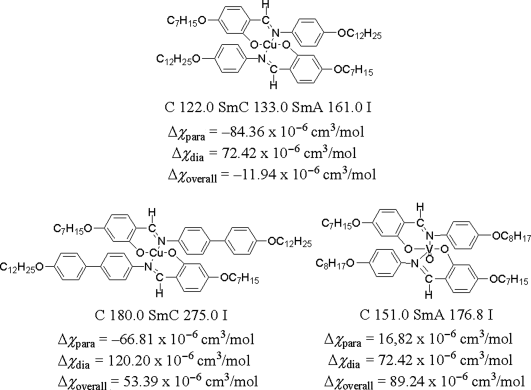
3.4.1.2 Intermolecular Magnetic Interactions
It should be emphasized that the McConnell type strong ferromagnetic interactions necessary for effective intermolecular spin–spin exchange are not expected for paramagnetic rod-like LC phases due to their dynamic behavior characterized by fast molecular rotation (1010–1011 s−1) around the molecular long axis [1], although theoretical considerations on the existence and dynamics of ferromagnetic LCs were presented [33, 34]. At present, no appreciable intermolecular ferromagnetic interaction has been observed in the LC state of rod-like d-block metallomesogens.
Interestingly, however, Jin et al. explicitly observed room temperature (RT) ferromagnetism generated in a diamagnetic discotic LC phase of tetra(2-decyltetradecyloxy)phthalocyanine derivative 6 intercalated with Fe(III)-phthalocyanine [Fe(III)Pc] over the range of magnetic field of ±2000 Oe (Figure 3.4), whereas Fe(III)Pc itself showed only a paramagnetic nature at RT. Outside of this range of magnetic field or at molar ratios of Fe(III)Pc to 6 of greater than 1/45, the hysteretic magnetization–magnetic field (M–H) curves characteristic of ferromagnetism disappeared and instead linear diamagnetic response was noted. The origin of the RT ferromagnetism observed in weak magnetic fields was ascribed to the magnetic interactions between the conductive spins in 6 and the localized spins in Fe(III)Pc in the LC state, based on EPR spectroscopy and SQUID magnetization measurements in the temperature range of 4.3–353 K [35].
Figure 3.4 LC columnar structure of diamagnetic 6 intercalated with Fe(III)Pc.
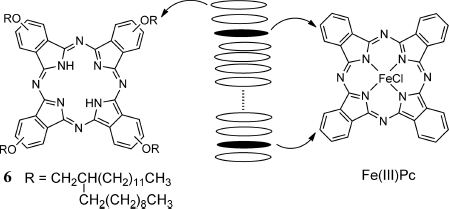
3.4.1.3 Spin Crossover
Spin crossover materials display labile electronic configurations switchable between the high spin and low spin states in response to external stimuli such as temperature, pressure, light, and magnetic field, showing distinct changes in magnetism, color, and structure [36]. The first single material combining spin crossover and LC behavior was a Fe(III) metallomesogen (low spin state S = 1/2; high spin state S = 5/2) exhibiting both properties not synchronously in different temperature intervals (Figure 3.5a) [37]. Later, synchronous spin crossover and LC transition have been devised for Fe(II) (S = 0; S = 2) (Figure 3.5b) [38] and Co(II) (S = 1/2; S = 3/2) metallomesogens (Figure 3.5c) [39].
Figure 3.5 Metallomesogens showing spin crossover: (a) Fe(III) (S = 1/2; S = 5/2), (b) Fe(II) (S = 0; S = 2), and (c) Co(II) (S = 1/2; S = 3/2).
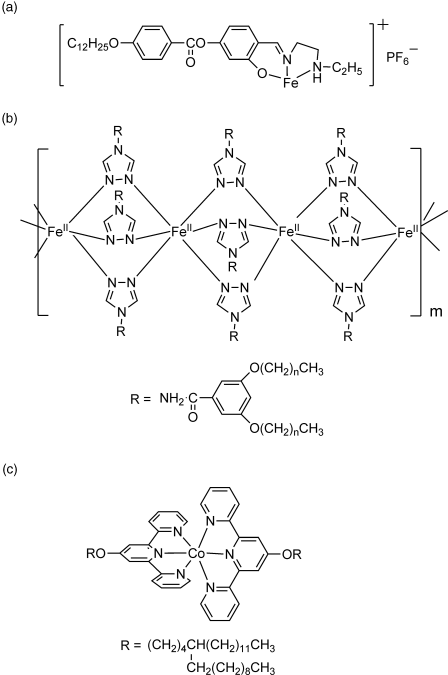
3.4.1.4 SMM
To fabricate SMMs with self-organized ordering, Rogez and Donnio et al. endowed the SMM Mn12 cluster molecule with LC properties [40]. The obtained two [Mn12O12L16(H2O)4] compounds which showed stable mesophases with 3D (cubic) or 1D (random mesh smectic) positional order between −11.5 and 150.0°C or between 40.5 and 150.0°C, respectively, behaved as SMMs with the blocking temperature below 8 K (Figure 3.6).
Figure 3.6 SMM Mn12 cluster molecules showing LC properties at high temperatures.
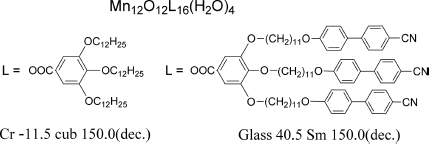
3.4.1.5 Ferroelectricity
To develop ferroelectric metallomesogens, several chiral transition metal-containing LC materials showing a SmC* phase were prepared. Among them, Cu(II) and VO(IV) metallomesogens with analogous chiral bis(salicylideneaniline) ligands were paramagnetic (Figure 3.7) [27, 29, 31]. However, they did not show any noteworthy optical and electric properties, compared with usual organic ferroelectric LCs. For example, the ferroelectric Cu(II)-mesogen 7 showed a spontaneous polarization Ps(−10°) of 38 nC cm−2 at 100°C and a long optical response time [τ(−10°)] of 8.5 ms, while its organic ligand itself exhibited better ferroelectricity with a slightly higher Ps(−10°) of 41 nC cm−2 and a shorter τ(−10°) of 1.1 ms than 7 [31]. No magnetic property was reported for these paramagnetic chiral metallomesogens.
Figure 3.7 Ferroelectric metallomesogen 7.
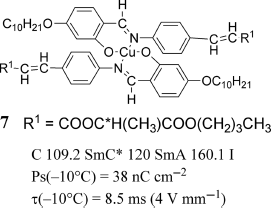
Meanwhile, chiral VO(IV) and Cu(II) β-diketone complexes 8 showed a RT columnar mesophase that underwent ferroelectric switching (Figure 3.8), although the Ps could not be evaluated [41, 42].
Figure 3.8 Switchable columnar metallomesogens 8.
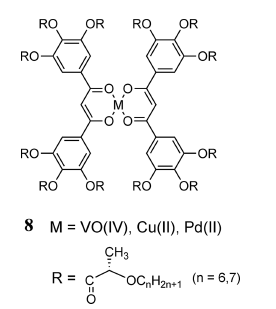
3.4.2 f-Block Metal Complexes
At present, no appreciable intermolecular ferromagnetic interaction has been observed in the LC state of f-block metallomesogens.
3.4.2.1 Magnetic Anisotropy
Not a few examples of rod-like metallomesogens containing 4f rare earth metal ions, such as Pr(III), Nd(III), Tb(III), Dy(III), and Er(III), have been documented (Figure 3.9) [24–26]. Due to the large anisotropy of the 4f orbital arising from the spin–orbital coupling, the magnitude of the Δχpara is as large as 810–5550 × 10−6 emu mol−1 and prevails over that of the Δχdia of organic ligands [24, 43–47]. Accordingly, the molecular alignment by magnetic fields should be Δχpara-controlled. However, the intrinsic high viscosity of rod-like f-block metallomesogens (SmA or columnar phases) brought about by the ligand-coordinated metal-complex structure generally renders the response to weak magnetic fields difficult and raises the phase transition temperature; a strong applied magnetic field (>1.2 T) is necessary even for the molecular alignment in the SmA phase during the cooling process from the isotropic phase and the crystal-to-LC phase transition generally occurs at a high temperature (>100°C). Therefore, these rod-like metallomesogens exhibiting SmA or columnar phases are not always appropriate materials for investigating the alignment of LC molecules by magnetic fields.
Figure 3.9 Rare earth metal-containing LCs and their magnetic anisotropy.
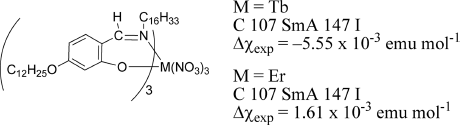
Recently Galyametdinov and Binnemans et al. reported the first example of lanthanidomesogens (La(III), Nd(III), Eu(III), and Yb(III)) that can exhibit a low viscous nematic (N) phase over a wide temperature range between ca. 100°C and ca. 140°C after the appearance of the SmA phase during the heating process (Figure 3.10). However, the electrical switching of the N phase of the pure lanthanide complex was not possible. The preparation of a lanthanide complex showing a stable N phase at room temperature is anticipated (see Section 3.4.2.2) [48].
Figure 3.10 Lanthanidomesogen 9 exhibiting an N phase.
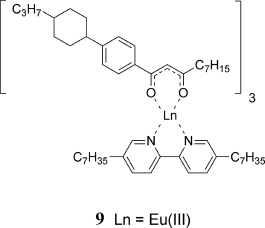
3.4.2.2 Luminescence
Galyametdinov and Binnemans et al. observed linearly polarized luminescence by exciting the homogeneously aligned SmA phase of the paramagnetic Eu(III) complex mesogen 9 in a 5 µm-thick sandwich cell with UV-irradiation (Figure 3.10) [48, 49]. However, the radiationless deactivation was dominant at the temperatures at which the N phase is stable (108–142°C). Therefore, it is anticipated that the linearly polarized luminescence can be observed in concert with electrical or magnetic switching of the aligned N phase of a pure lanthanide complex at room temperature.
3.4.2.3 SMM
Veciana et al. prepared a SMM with two different relaxation processes by controlling the cooling rate from the LC phase of a double-decker terbium complex 10 (Figure 3.11) [20].
Figure 3.11 SMM double-decker terbium complex 10 showing LC properties at high temperatures.
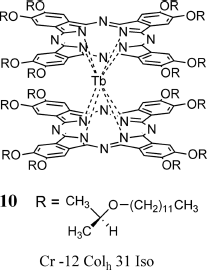
3.5 All-Organic Radical LCs
All-organic rod-like LC materials with a stable radical unit, which can benefit from the low viscosity and small molecular size, may show unique intermolecular magnetic interactions owing to the swift coherent collective properties of organic molecules in the LC state, although their Δχpara is considered to be too small to control the molecular orientation by magnetic fields due to the p-orbital origin.
3.5.1 First-Generation of Rod-Like All-Organic Radical LCs
Only a few all-organic radical LC compounds have been prepared, most likely because the geometry and bulkiness of the radical-stabilizing substituents are detrimental to the stability of LCs, which requires molecular linearity or planarity [1]. Although several achiral rod-like organic LCs with a stable nitroxyl group (DOXYL or TEMPO group) as the spin source were prepared (Figure 3.12), their molecular structures were limited to those containing the nitroxyl group within the terminal alkyl chain, away from the rigid core, and hence allowed the free rotation of the nitroxyl moiety inside the molecule, resulting in a decrease in the Δχpara as well as the Δε of the whole molecule. All attempts to prepare monomeric or polymeric LC compounds with a nitroxyl unit as part of the rigid core were unsuccessful. The molecular structures and magnetic properties of such first-generation of all-organic nitroxyl radical LCs and the objectives of individual studies are briefly summarized below.
Figure 3.12 First-generation of rod-like all-organic radical LC compounds 11–17.
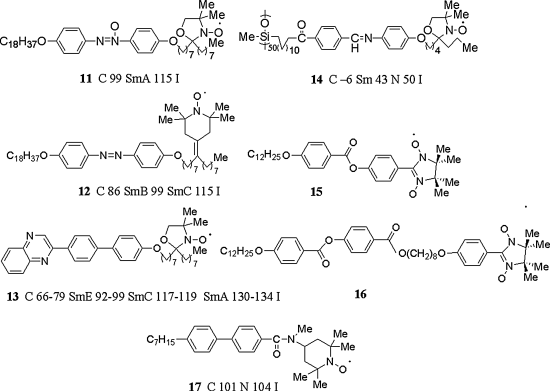
Dvolaitzky et al. synthesized chiral racemic and achiral compounds 11–13 to use them as a LC spin-probe for EPR spectroscopic study. Racemic 13 showed stable smectic phases such as SmA, SmC, and SmE [50–52]. Their temperature dependence of the χ was not measured.
With the aim of measuring the magnetic properties of a LC structure at low temperatures, Finkelmann et al. prepared chiral racemic radical polymer 14 which can retain the LC structure in the supercooled glassy phase [53]. By means of a Faraday balance, the temperature dependence of the χ was measured in the temperature range from 6 to 350 K, in which the crystal-to-LC-to-liquid phase transition occurred. Consequently, during the heating process in this temperature range, 14 showed no molecular orientation; no appreciable change in χ was observed at the crystal-to-LC phase transition temperature. This is most likely due to the high viscosity of the polymer material. It is desirable to measure the temperature dependence of the χ during the cooling process from the isotropic phase on a SQUID susceptometer.
Greve et al. synthesized the first LC compounds 15 and 16 bearing an α-nitronyl nitroxide (α-NN) structure as a spin source at the terminal position in the molecule [54]. They showed a highly viscous monotropic LC phase in the narrow temperature range from 36 to 39°C during the heating process. The temperature dependence of the χ was not measured.
To prepare a supercooled glassy material and crystal polymorphs in the applied magnetic fields and to observe the change in the magnetic behavior accompanying the alteration in the solid-state structure, achiral LC compound 17 was synthesized by Nakatsuji et al. [19]. Although 17 showed the N phase within a narrow temperature range of 3° during the heating process, a distinct increase in χ was observed at the crystal-to-LC transition temperature. The difference in the magnetic behavior between the heating and cooling processes was also observed; 17 showed antiferromagnetic interactions according to a singlet–triplet model at low temperatures before the thermal phase transition during the heating process of the crystals, while the magnetic behavior obeyed the Curie–Weiss law during the cooling process from the isotropic phase (Eq. (3.8)).
where C is the Curie constant, T is the temperature, and θ is the Weiss temperature.
3.5.2 Discotic All-Organic Radical LCs
Use of π-delocalization of an unpaired electron is an alternative method to stabilize the radical species. Based on this methodology, the cation-radical charge-transfer salts 18a and 18b with semiconductivity were prepared from the corresponding tetraphenylbipyranylidene or tetraphenylbithiopyranylidene derivative and tetracyanoquinodimethane (TCNQ) by Saeva et al. and Strzelecka et al., respectively (Figure 3.13) [55, 56]. The salt 18a showed a discotic LC phase over a wider temperature range and a lower clearing point than the neutral tetraphenylbipyranylidene, while the salt 18b showed a SmA phase. The magnetic susceptibility measurement of 18b by the Faraday method indicated that there were no interacting spins between the donor and the acceptor in the LC phase, while the weak antiferromagnetic behavior characteristic of interacting spins was observed below the crystal-to-LC transition point of 120°C.
Figure 3.13 LC charge-transfer complexes 18.
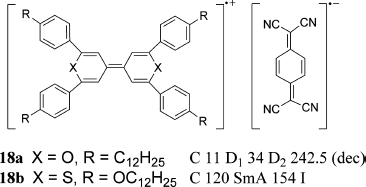
Nakatsuji et al. successfully prepared the first stable discotic all-organic radical LC compounds 19 and 20, which have a nitroxide unit (TEMPO or PROXYL group) at the peripheral position in the molecule and showed an enantiotropic columnar phase over a wide temperature range (Figure 3.14). Weak antiferromagnetic interactions were observed in the crystalline, LC, and supercooled phases by measuring the temperature dependence of the χ in the temperature range between 2 and 300 K [57].
Figure 3.14 Discotic nitroxide radical LC compounds 19 and 20.
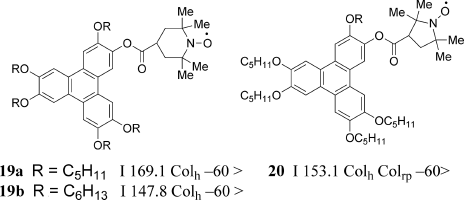
Recently, Julia and Velasco et al. successfully synthesized the discotic radical compound 21, which has a stable tris(2,4,6-trichlorophenyl)methyl radical structure as a spin source in the core of LC molecule (Figure 3.15). They observed distinct antiferromagnetic interactions in the columnar LC phases by measuring the temperature dependence of the χ in the temperature range between 2 and 400 K [58].
Figure 3.15 Discotic triphenylmethyl radical LC compound 21.
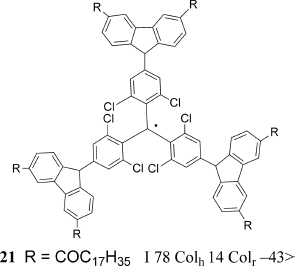
3.5.3 Second-Generation of Rod-Like All-Organic Radical LCs
3.5.3.1 Molecular Design and Synthesis
To examine the several possibilities anticipated for PLCs in Section 3.1, the second-generation of chiral nitroxide molecules that could satisfy the following four mandatory requirements were designed.
Spin source: Among persistent or stable organic free radicals such as nitroxides [59], verdazyls [60, 61], thioaminyls [62], a certain hydrazyl [63], phenoxyls [64, 65], and carbon-centered radicals [58, 66], a nitroxyl group, which serves as the spin source of nitroxides and possesses a large electric dipole moment (ca. 3 Debye) and known principal g-values (gxx, gyy, gzz) should be the best spin source, because (i) the dipole moment is large enough for the source of the Ps and (ii) the principal g-values are useful to determine the direction of molecular alignment in the LC phase by EPR spectroscopy (Figure 3.16a).
Figure 3.16 Principal g-values of a nitroxide molecule.
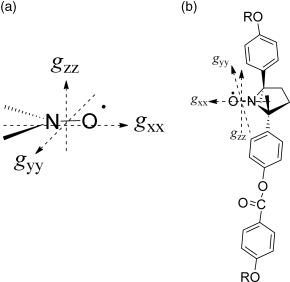
High thermal stability: A 2,2,5,5-tetraalkyl-substituted pyrrolidine-1-oxy (PROXYL) structure is stable enough for repeated heating and cooling cycles below 150°C in the air.
Molecular structure:
(a) To avoid the free rotation of the nitroxyl group inside the molecule so as to maximize the Δχpara and Δε, a geometrically fixed chiral cyclic nitroxide unit should be incorporated into the rigid core of LC molecules. (b) To obtain a slightly zigzag molecular structure and a negative Δε advantageous for the appearance of a SmC* phase, a trans-2,5-dimethyl-2,5-diphenylpyrrolidine-1-oxy (PROXYL) skeleton in which the electric dipole moment orients to the molecular short axis is the best choice (Figure 3.16b).Chirality: Since both chiral and achiral LCs are required for comparison of their optical and magnetic properties in various phases, the molecules should be chiral and both enantiomerically enriched and racemic samples need to be available.
According to the above requirements, structurally different five LC compounds (1–5) with a negative Δε were prepared in both enantiomerically enriched and racemic forms (Figure 3.1) [5–7, 16, 67–70].
3.5.3.2 Characterization of LC Phases
Individual LC phase types of compounds 1–5 were determined by means of differential scanning calorimetry (DSC), hot-stage polarized optical microscopy (POM), and variable temperature X-ray diffraction (VT-XRD) analysis under random or homogeneous planar boundary conditions [16, 67–70].
Compounds 1–4 showed chiral (or achiral) N* (N) and/or SmC* (SmC) phases (Figure 3.17a) [16, 67, 68], while compounds 5 possessing the partially modified molecular structures of 1 exhibited a different sequence of LC phases, N* (N), SmA* (SmA) and SmC* (SmC) (Figure 3.17b) [69, 70]. This difference in the LC phase change can be explained in terms of the difference in the direction of molecular dipole moment between 1 and 5 [70]. It is noteworthy that compounds 1 showed the LC phases over a wide range of temperature between ambient temperature and 90°C, particularly on the cooling run.
Figure 3.17 LC behavior of (a) (2S,5S)-1 and (b) (2S,5S)-5.
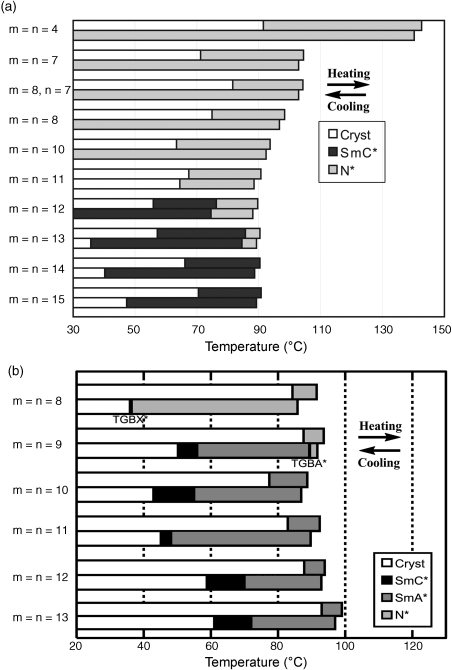
3.5.3.3 Magnetic Properties
Since the magnetic properties such as Δχ- controlled molecular orientation and magnetic interactions in all-organic PLC phases have been fully characterized for the first time by using the various LC phases of compounds 1, these experimental results are described in detail.
Magnetic Anisotropy and Magnetic-Field-Induced Molecular Alignment
In Section 3.4, it has been stated that rod-like metallomesogens with high viscosity are not always appropriate for the investigation on the alignment of LC molecules by magnetic fields. In contrast, LC compounds 1 with low viscosity, low phase transition temperature, and known principal g-values of the nitroxide moiety are considered to be a good candidate for the studies on the Δχ-controlled molecular orientation by weak magnetic fields, albeit its small Δχpara. Therefore, we determined whether the magnetic-field-induced molecular alignment in the LC phases of 1 was dominated by the Δχpara or Δχdia, by the quantitative evaluation of the Δχpara and Δχdia values of 1 and then evaluated the approximate magnitude of Hc for each LC phase of 1 by EPR spectroscopy and by POM observation under variable magnetic fields [71].
First, the temperature-dependent Δχpara value of compound 1a (m = n = 13) was calculated to be −1.7 × 10−6 emu mol−1 at 300 K from the g-value obtained by EPR spectroscopy, while the temperature-independent Δχdia value was calculated to be +6.5 × 10−5 emu mol−1 from the experimental molar magnetic susceptibility of (±)-1a measured on a SQUID magnetometer. Thus, χdia
has turned out to be 30 times larger than
χpara
; the molecular alignment of 1a by magnetic field is definitely Δχdia-controlled.
Next, to identify the direction of molecular alignment in the bulk LC state under a weak magnetic field, the temperature dependence of the experimental g-value (gexp) of (±)-1a was measured at a magnetic field of 0.33 T by EPR spectroscopy (Figure 3.18). During the heating process, the gexp of (±)-1a was constant at around 2.0065 in the crystalline state, then increased at the crystal-to-SmC phase transition, became constant at around 2.0068 in the SmC phase, then decreased abruptly to 2.0058 at the SmC-to-N phase transition, and finally returned to the level (~2.0065) of the crystalline state in the isotropic phase. During the cooling process, the gexp of (±)-1a was constant at around 2.0065 in the isotropic phase, then decreased at the Iso-to-N phase transition, became constant at around 2.0055 in the N phase, then increased to 2.0063 at the N-to-SmC phase transition, and finally increased to 2.0067 in the crystalline state.
Figure 3.18 EPR spectroscopy of (±)-1a (m = n = 13). (a) Experimental setup and (b) temperature dependence of the g-value measured through the first heating (white circles) and cooling (black circles) processes. Source: Ref. [71] – Reproduced by permission of The Royal Society of Chemistry.
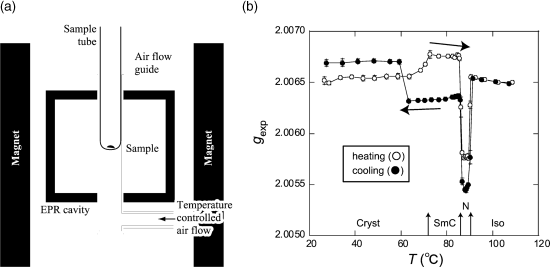
From these results and the calculated principal g-values (giso = 2.00632, g = 2.00540, g⊥ = 2.00678) of 1a, it is concluded that (i) in the N phase the majority of molecules align their long axis along the applied magnetic field of 0.33 T (Figure 3.19a), whereas in the SmC phase during the heating process the molecular short axis is almost parallel to the field (Figure 3.19b), most likely due to the viscous layer structure and the natural homeotropic anchoring effect by quartz surface, and (ii) that the molecular alignment in each LC phase is influenced by that in the preceding LC phase, although the molecular orientation modes are quite different between the N and SmC phases.
Figure 3.19 Molecular alignment in the LC phases of (±)-1a under a weak magnetic field (0.34 T). (a) N phase during both heating and cooling processes and (b) SmC phase during the heating process.
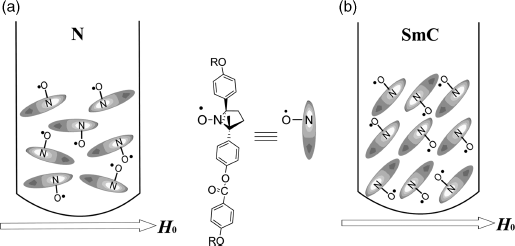
To evaluate the Hc for each LC phase of (±)-1a, we observed the texture change by POM observation under variable magnetic fields. Figure 3.20 shows the experimental setup. The direction of applied magnetic fields is perpendicular to the LC cell surface. The inner glass surface in the sandwich cell with 40 µm thickness was neither chemically nor physically treated. The random texture of the N phase gradually became dark with the increasing magnetic field until 0.5 T, resulting in the complete homeotropic orientation of molecules at 1.0 T, whereas the random texture of SmC phase scarcely changed below 1.0 T, largely changed between 1.0 T and 1.5 T, and finished the change at less than 2.0 T to show a Schlieren texture [71], which is similar to the SmC Schlieren texture of (±)-1 observed under alternative homeotropic boundary conditions [14]. Accordingly, it has been concluded that the smectic layer planes became parallel to the glass plates at 2.0 T. Furthermore, no texture change was noted for N* and SmC* phase of (2S,5S)-1a below 5 T using the same experimental setup.
Figure 3.20 Experimental setup for the polarized optical microscopy observation of (±)-1a under variable magnetic fields applied perpendicular to the cell surface. Source: Ref. [71] – Reproduced by permission of The Royal Society of Chemistry.
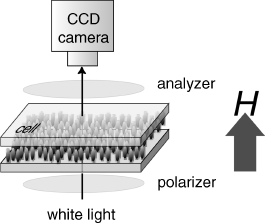
Thus, the Hc of each LC phase turned out to be largely affected by the superstructure; Hc(N) (<1.0 T) < Hc(SmC) (<2.0 T) < Hc(N*, SmC*) (>5.0 T).
Inhomogeneous Intermolecular Magnetic Interactions
As mentioned in Section 3.4.1.2, the possibility of a ferromagnetic rod-like LC material has been considered unrealistic due to the inaccessibility of long-range spin–spin interactions between rotating molecules in the LC state. Nevertheless, it is interesting to know whether and how LC domains can help to induce magnetic interactions in low viscous all-organic radical LC phases in which order coexists with fluidity.
Interestingly, we observed a nonlinear relationship (S-curve) between the applied magnetic field (H) and the observed magnetization (M) in chiral and achiral LC phases of 1 (Figure 3.21), which implies the generation of an unusual magnetic interaction in the LC phases under applied magnetic fields [17, 18]. Such a nonlinear paramagnetic magnetization (NPM) was not observed in the crystalline phases of the same compounds, which showed a usual linear relationship indicating a paramagnetic nature and no contamination of magnetic impurities in the sample. The in-depth investigation on the magnetic properties of LC compounds 1 strongly suggested that the generation of a sort of spin glass (SG)-like inhomogeneous magnetic interactions (the average spin–spin interaction constant ) induced by weak magnetic fields in the various LC phases of compounds 1 is responsible for the observed NPM; the magnitude of magnetic interactions depended on the LC phase type, or the superstructure (Figure 3.22) [18]. Furthermore, it was concluded that (i) the molecular reorientation effect arising from the simple molecular magnetic anisotropy (Δχ) has nothing to do with the NPM or the unusual magnetic interactions observed in the LC phases of 1 and instead (ii) the origin of such strong SG-like inhomogeneous magnetic interactions, which has been referred to as positive ‘magneto-LC effects’, can be interpreted in terms of the anisotropic spin–spin dipole interactions induced in the anisotropic LC superstructure under magnetic fields.
Figure 3.21 Magnetic field (H) dependence of molar magnetization (M) at 77°C for (a) the SmC phase of (±)-1a, (b) the N phase of (±)-1b (m = n = 8), (c) the SmC* phase of (2S,5S)-1a (88% ee), and (d) the N* phase of (2S,5S)-1b (96% ee). Source: Ref. [18] – Reprinted with permission from the American Chemical Society.
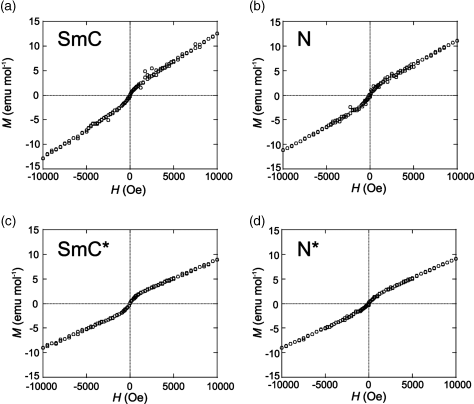
Figure 3.22 Temperature dependence of relative paramagnetic susceptibility (χrel) for (a) (±)-1a, (b) (±)-1b, (c) (2S,5S)-1a (88% ee), and (d) (2S,5S)-1b (96% ee) at a magnetic field of 0.33 T. Open and filled circles represent the first heating and cooling runs, respectively. The LC temperatures shown in a box refer to the first heating process. Source: Ref. [18] – Reprinted with permission from the American Chemical Society.
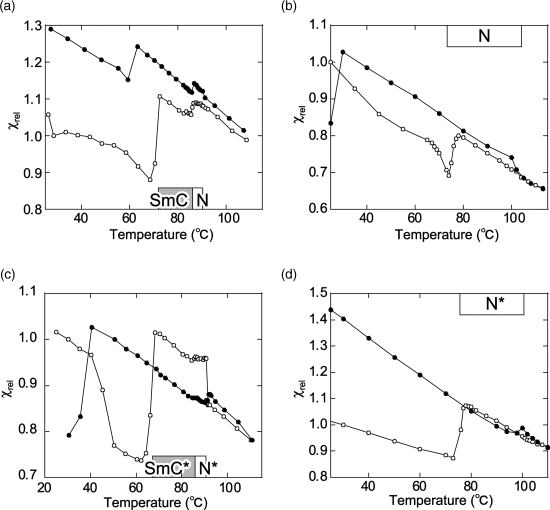
In this study, we could indicate that EPR spectroscopy is the much better means than SQUID magnetization measurement to measure the temperature dependence of the χpara for organic nitroxide radical LC materials at high temperatures. This is due to the following four reasons: (i) The χpara can be derived from the Bloch equation (Eq. (3.9)) by using the parameters obtained from the EPR differential curves, such as maximum peak height ( and −
), g-value (g), and peak-to-peak line width (ΔHpp).
where μB is the Bohr magnetron, h is Planck's constant, ν is the frequency of the absorbed electromagnetic wave, and H1 is the amplitude of the oscillating magnetic field. Accordingly, the temperature dependence of relative paramagnetic susceptibility (χrel), which is defined as
where χ0 is the standard paramagnetic susceptibility, e.g., at 30°C in the heating run (Eq. (3.10)), can be actually used (Figure 3.22). (ii) Treatment of the χdia term is totally unnecessary. (iii) The experimental error is very small even at such high temperatures. (iv) The analysis of microscopic magnetic interactions such as spin–spin dipole and exchange interactions is also feasible.
Furthermore, these radical LC droplets floating on water were uniformly attracted by a permanent magnet and moved freely on water under the influence of this magnet (Figure 3.23), whereas the crystallized particles of the same compounds never responded to the same magnet. The response of the LC droplets to the magnet also varied depending on the LC phase type, that is, the extent of the magnetic interaction (). These results indicate that the LC phase domain can help to induce magnetic interactions under applied magnetic fields [17, 18].
Figure 3.23 Schematic representation of the experimental setup for observing the attraction by a permanent magnet (maximum 0.5 T) of a paramagnetic LC droplet on water in a shallow laboratory dish. Source: Ref. [18] – Reprinted with permission from the American Chemical Society.
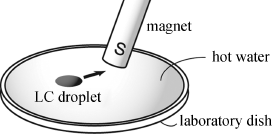
This unique magnetic attraction will find use in the development of the metal-free magnetic soft materials usable at ambient temperature, such as a magnetic LC carrier for the magnetically targeted drug-delivery system or an MRI contrast agent [72].
3.5.3.4 Ferroelectricity
It is known that when a SmC* phase is confined to a thin sandwich cell with a gap smaller than the pitch of the helical superstructure, an unwinding of the helix occurs and a bistable, ferroelectric device is formed (Figure 3.24) [14, 73]. Consequently, Ps is generated in the sandwich cell in which ferroelectric switching occurs by changing the polarity of the electric field. The ferroelectric properties of the SmC* phase of each sample are characterized by measuring the Ps, the optical response time of bistable switching to an applied electric field, and the tilt angle.
Figure 3.24 Ferroelectric switching in a thin sandwich cell.
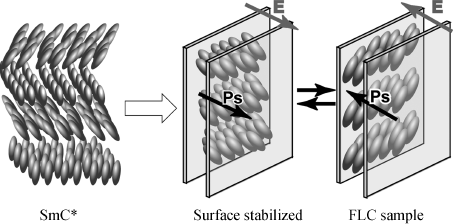
The SmC* phases of (2S,5S)-1–5 indeed exhibited ferroelectricity in a planar anchoring thin sandwich cell (4 µm thickness) (Table 3.1) [67–70]. The ferroelectric properties of (2S, 5S)-1 deserve attention; e.g., for (2S, 5S)-1a, a Ps value of 24 nC cm−2, an optical response time (τ10–90) of 0.213 ms and a layer tilt angle (θ) of 29° were recorded. The FLC data of (2S, 5S)-1a were superior to those of (2S, 5S)-2–5 [67–70] and the typical chiral metallomesogen 7 (Figure 3.7); 7 showed a higher Ps value of 38 nC cm−2 with a θ of 23° than (2S, 5S)-1a, but the optical response was very slow (τ10–90 = 8.5 ms) due to the high viscosity (Table 3.1) [31].
Table 3.1 Comparison of ferroelectric properties.
Furthermore, second-harmonic generation (SHG) was clearly observed by Kogo and Takezoe et al. under a phase-matching condition in the SmC* phase of (2S,5S)-1a loaded into an LC cell (20 µm thickness), validating the existence of ferroelectricity. The effective second-order nonlinear optical (NLO) constant was evaluated to be a 4.8 × 10−2 pm V−1, 3 orders of magnitude smaller than that of quartz known as a standard NLO material [74].
3.6 Conclusions
The magnetic properties of two different types of paramagnetic LC materials, d- and f-block metallomesogens and all-organic radical LCs, were briefly surveyed.
For objective 1 listed in Section 3.1, lanthanide complexes showing a stable N phase at room temperature is most promising and their emergence is strongly anticipated.
In the context of objectives 2–4, noteworthy is the first observation of unique SG-like inhomogeneous intermolecular magnetic interactions () in the LC state under weak magnetic fields of the second-generation of rod-like all-organic chiral radical LC compounds 1 that can show thermally stable chiral and achiral rod-like LC phases such as N* and/or SmC* and N and/or SmC phases, respectively. Furthermore, the ferroelectric properties of the SmC* phases of 1 were fully characterized in a sandwich cell; a nitroxyl group proved to act as the sufficient source of the macroscopic electric dipole that can be evaluated as Ps. Such chiral all-organic radical LCs with low viscosity showed faster ferroelectric switching than chiral metallomesogens with high viscosity. The next step is the control of magnetic properties of ferroelectric PLCs of 1 by electric fields (and vice versa). In addition, it is of great advantage to use EPR spectroscopy and SQUID magnetization measurement as the tool for observing and evaluating the microscopic and macroscopic dynamic behaviors of the LC materials, respectively. Particularly, EPR spectroscopy has proved to be an excellent tool in place of SQUID magnetization measurement for measuring the temperature dependence of the χpara for organic nitroxide radical LC phases at high temperatures. Thus, such second-generation of all-organic chiral radical LC compounds would open up a new research field of LC materials.
In terms of objective 3, another notable finding is the observation of RT ferromagnetism in a diamagnetic discotic LC phase of 6 intercalated with paramagnetic Fe(III)Pc under weak magnetic fields. This result would provide general guidelines for the fabrication of ferromagnetic discotic LCs.
As far as objectives 6 and 7 are concerned, the molecular design of sophisticated metallomesogens aiming for each desired function would be necessary.
The research on PLCs is in its infancy. Hence, PLCs would gradually become fascinating dynamic magnetic functional materials as these objectives can be achieved step-by-step. Therefore, it is envisaged that sooner or later PLCs would emerge as an important subfield of liquid crystal science at its own right.
Acknowledgments
We acknowledge Professor Takeji Takui, Professor Hiroyuki Nohira, Dr. Yoshio Aoki, Professor Hideo Takezoe, Dr. Yoshio Shimbo, Ms. Reiri Kogo, Professor Jun Yamauchi, Dr. Yohei Noda, Dr. Naohiko Ikuma and Dr. Satoshi Shimono for their collaboration.
1. P. Kaszynski. Liquid crystalline radicals: an emerging class of organic magnetic materials. In: P. M. Lahti, Ed., Magnetic Properties of Organic Materials, Marcel Dekker, New York, 1999.
2. K. Griesar and W. Haase. Magnetic properties of transition-metal-containing liquid crystals. In: P. M. Lahti, Ed., Magnetic Properties of Organic Materials, Marcel Dekker, New York, 1999.
3. D. Dunmur and K. Toriyama. Magnetic properties of liquid crystals. In: D. Demus, J. Gooby, G. W. Gray, and S. V. Vill, Eds., Physical Properties of Liquid Crystals, Wiley-VCH, Weinheim, 1999.
4. J.-L. Serrano. Metallomesogens: Synthesis, Properties, and Applications, Wiley-VCH, Weinheim, 1996.
5. R. Tamura. Organic functional materials containing chiral nitroxide radical units. In: G. I. Likhtenshtein, J. Yamauchi, S. Nakatsuji, A. I. Smirnov, and R. Tamura, Eds., Nitroxides: Application in Chemistry, Biomedicine, and Materials Science, Wiley-VCH, Weinheim, 2008.
6. R. Tamura, Y. Uchida, and N. Ikuma. Paramagnetic all-organic chiral liquid crystals. J. Mater. Chem. 2008, 2008, 2872–2876.
7. R. Tamura, N. Ikuma, and S. Shimono. Preparation and magnetic properties of all-organic radical liquid crystals. In: H. S. Nalwa, Ed., Soft Nanomaterials. Volume 1, American Scientific Publishers, CA, 2009.
8. W. Eerenstein, N. D. Mathur, and J. F. Scott. Multiferroic and magnetoelectric materials. Nature 2006, 2006, 759–765.
9. C. N. R. Rao and C. R. Serrao. New routes to multiferroics. J. Mater. Chem. 2007, 2007, 4931–4938.
10. C. Felser, G. H. Fecher, and B. Balke. Spintronics: a challenge for materials science and solid-state chemistry. Angew. Chem. Int. Ed. 2007, 2007, 668–699.
11. G. L. J. A. Rikken and E. Raupach. Observation of magneto–chiral dichroism. Nature 1997, 1997, 493–494.
12. G. L. J. A. Rikken and E. Raupach. Enantioselective magnetochiral photochemistry. Nature 2000, 2000, 932–935.
13. C. Train, R. Gheorghe, V. Krstic, L.-M. Chamoreau, N. S. Ovanesyan, G. L. J. A. Rikken, M. Gruselle, and M. Verdaguer. Strong magneto–chiral dichroism in enantiopure chiral ferromagnets. Nature Mater. 2008, 7, 729–734.
14. I. Dierking. Textures of Liquid Crystals, Wiley-VCH, Weinheim, 2003.
15. D. Dunmur and K. Toriyama. Elastic properties. In: D. Demus, J. Gooby, G. W. Gray, and S. V. Vill, Eds., Physical Properties of Liquid Crystals, Wiley-VCH, Weinheim, 1999.
16. N. Ikuma, R. Tamura, S. Shimono, N. Kawame, O. Tamada, N. Sakai, J. Yamauchi, and Y. Yamamoto. Magnetic properties of all-organic liquid crystals containing a chiral five-membered cyclic nitroxide unit within the rigid core. Angew. Chem. Int. Ed. 2004, 2004, 3677–3682.
17. Y. Uchida, N. Ikuma, R. Tamura, S. Shimono, Y. Noda, J. Yamauchi, Y. Aoki, and H. Nohira. Unusual intermolecular magnetic interaction observed in an all-organic radical liquid crystal. J. Mater. Chem. 2008, 2008, 2950–2952.
18. Y. Uchida, K. Suzuki, R. Tamura, N. Ikuma, S. Shimono, Y. Noda, and J. Yamauchi. Anisotropic and inhomogeneous magnetic interactions observed in all-organic nitroxide radical liquid crystals. J. Am. Chem. Soc. 2010, 2010, 9746–9752.
19. S. Nakatsuji, M. Mizumoto, H. Ikemoto, H. Akutsu, and J. Yamada. Preparation and properties of organic radical compounds with mesogenic cores. Eur. J. Org. Chem. 2002, 1912–1918.
20. M. Gonidec, F. Luis, A. Vilchez, J. Esquena, D. B. Amabilino, and J. Veciana. A liquid-crystalline single-molecule magnet with variable magnetic properties. Angew. Chem. Int. Ed. 2010, 2010, 1623–1626.
21. H. J. Müller and W. Haase. Magnetic susceptibility and the order parameters of some 4,4′-disubstituted biphenyl cyclohexanes. J. Phys. (Paris) 1983, 1983, 1209–1213.
22. M. I. Boamfa, M. W. Kim, J. C. Maan, and T. Rasing. Observation of surface and bulk phase transitions in nematic liquid crystals. Nature 2003, 2003, 149–152.
23. S. A. Hudson and P. M. Maitlis. Calamitic metallomesogens: metal-containing liquid crystals with rodlike shapes. Chem. Rev. 1993, 1993, 861–885.
24. K. Binnemans and C. Gröller-Walrand. Lanthanide-containing liquid crystals and surfactants. Chem. Rev. 2002, 2002, 2303–2345.
25. E. Terazzi, S. Suarez, S. Torelli, H. Nozary, D. Imbert, O. Mamula, J.-P. Rivera, E. Guillet, J.-M. Benech, G. Bernardinelli, R. Scopelliti, B. Donnio, D. Guillon, J.-C. G. Bunzli, and C. Piguet. Introducing bulky functional lanthanide cores into thermotropic metallomesogens: a bottom-up approach. Adv. Funct. Mater. 2006, 2006, 157–168.
26. C. Piguet, J.-C. G. Bunzli, B. Donnio, and D. Guillon. Thermotropic lanthanidomesogens. Chem. Commun. 2006, 3755–3768.
27. M. Marcos and J. L. Serrano. Paramagnetic nematic liquid crystals. Adv. Mater. 1992, 1992, 256–257.
28. M. Maracos, J. L. Serrano, T. Sierra, and M. J. Gimenez. Paramagnetic chiral smectic C materials: a new class of ferroelectric liquid crystals. Angew. Chem. Int. Ed. 1992, 1992, 1471–1472.
29. K. Griesar, Y. Galyametdinov, M. Athanassopoulou, I. Ovchinnikov, and W. Haase. Paramagnetic liquid crystalline nickel(II) compounds. Adv. Mater. 1994, 1994, 381–384.
30. P. J. Alonso, M. Maarcos, J. Martinez, J. L. Serrano, and T. Sierra. EPR study of a chiral metallomesogens: bis(N- [4″-((2S)-2-chloropropoxy)phenyl], 4-(4′-n-decyloxybenzoyloxy)salicylaldimine)copper(II). Adv. Mater. 1994, 1994, 667–670.
31. R. Iglesias, M. Marcos, J. L. Serrano, T. Sierra, and M. A. Perez-Jubindo. Ferroelectric behavior of chiral bis(salicylideneaniline) copper(II), vanadium(IV), and palladium(II) liquid crystals. Chem. Mater. 1996, 1996, 2611–2617.
32. I. B. Yu, Y. Galyametdinov, A. Prosvirin, K. Griesar, E. A. Soto-Bustamante, and W. Haase. Correlation between magnetic properties and molecular structure of some metallo-mesogens. Liq. Cryst. 1995, 1995, 231–237.
33. M. Buivydas. Some conditions of ferromagnetic liquid crystal existence. Phys. Status Solidi B 1991, 1991, 577–581.
34. E. I. Kats and V. V. Lebedev. Some properties of ferromagnetic liquid crystals. Mol. Cryst. Liq. Cryst. 1991, 1991, 329–337.
35. C. H. Lee, Y.-W. Kwon, D. H. Choi, Y. H. Geerts, E. Koh, and J.-I. Jin. High-temperature ferromagnetism of a discotic liquid crystal dilutely intercalated with Iron(III) phthalocyanine. Adv. Mater. 2010, 2010, 4405–4409.
36. A. B. Gaspar, M. Seredyuk, and P. Gütlich. Spin crossover in metallomesogens. Coord. Chem. Rev. 2009, 2009, 2399–2413.
37. Y. Galyametdinov, V. Ksenofontov, A. Prosvirin, I. Ovchinnikov, G. Ivanova, P. Gütlich, and W. Haase. First example of coexistence of thermal spin transition and liquid-crystal properties. Angew. Chem. Int. Ed. 2001, 2001, 4269–4271.
38. T. Fujigaya, D.-L. Jiang, and T. Aida. Switching of spin states triggered by a phase transition: spin-crossover properties of self-assembled iron (II) complexes with alkyl-tethered triazole ligands. J. Am. Chem. Soc. 2003, 2003, 14690–14691.
39. S. Hayami, R. Moriyama, A. Shuto, Y. Maeda, K. Ohta, and K. Inoue. Spin transition at the mesophase transition temperature in a cobalt (II) compound with branched alkyl chains. Inorg. Chem. 2007, 2007, 7692–7694.
40. E. Terazzi, C. Bourgogne, R. Welter, J.-L. Gallani, D. Guillon, G. Rogez, and B. Donnio. Single-molecule magnets with mesomorphic lamellar ordering. Angew. Chem. Int. Ed. 2008, 2008, 490–495.
41. J. Barberá, R. Iglesias, J. L. Serrano, T. Sierra, M. R. de la Fuente, B. Palacios, M. A. Pérez-Jubindo, and J. T. Vázquez. Switchable columnar metallomesogens. New helical self-assembling systems. J. Am. Chem. Soc. 1998, 1998, 2908–2918.
42. J. L. Serrano and T. Sierra. Switchable columnar metallomesogens. Chem. Eur. J. 2000, 2000, 759–766.
43. Y. Galyametdinov, M. A. Athanassopoulou, K. Griesar, O. Kharitonova, E. A. Soto-Bustamante, L. Tinchurina, I. Ovchinnikov, and W. Haase. Synthesis and magnetic investigation on rare-earth-containing liquid crystals with large magnetic anisotropy. Chem. Mater. 1996, 1996, 922–926.
44. K. Binnemans, Y. G. Galyametdinov, R. Van Deun, D. W. Bruce, S. R. Collinson, A. P. Polishchuk, I. Bikchantaev, W. Haase, A. V. Prosvirin, L. Tinchurina, I. Litvinov, A. Gubajdullin, A. Rakhmatullin, K. Uytterhoeven, and L. Van Meervelt. Rare-earth-containing magnetic liquid crystals. J. Am. Chem. Soc. 2000, 2000, 4335–4344.
45. V. S. Mironov, Y. G. Galyametdinov, A. Ceulemans, and K. Binnemans. On the magnetic anisotropy of lanthanide-containing metallomesogens. J. Chem. Phys. 2000, 2000, 10293–10303.
46. Y. G. Galyametdinov, W. Haase, L. Malykhina, A. Prosvirin, I. Bikchantaev, A. Rakhmatullin, and K. Binnemans. Synthesis, mesomorphism, and unusual magnetic behavior of lanthanide complexes with perfluorinated counterions. Chem. Eur. J. 2001, 2001, 99–105.
47. Y. G. Galyametdinov, W. Haase, B. Gorderis, D. Moors, K. Driesen, R. Van Deun, and K. Binnemans. Magnetic alignment study of rare-earth-containing liquid crystals. J. Phys. Chem. B 2007, 2007, 13881–13885.
48. Y. G. Galyametdinov, A. A. Knyazev, V. I. Dzhabarov, T. Cardinaels, K. Driesen, C. Görller-Walrand, and K. Binnemans. Polarized luminescence from aligned samples of nematogenic lanthanide complexes. Adv. Mater. 2008, 2008, 252–257.
49. K. Binnemans. Lanthanide-based luminescent hybrid materials. Chem. Rev. 2009, 2009, 4283–4374.
50. M. Dvolaitzky, J. Billard, and F. Poldy. Notes des Membres et Correspondants et Notes Présentées ou Transmises par Leurs Soins, C. R. Acad. Sci. 1974, 279C, 533–535.
51. M. Dvolaitzky, C. Taupin, and F. Poldy. Nitroxides piperidiniques – Synthese de nouvelles sondes paramagnetiques. Tetrahedron Lett. 1976, 18, 1469–1472.
52. M. Dvolaitzky, J. Billard, and F. Poldy. Smectic E, C and A Free Radicals, Tetrahedron 1976, 1976, 1835–1838.
53. J. Allgaier and H. Finkelmann. Synthesis and magnetic properties of mesogenic side-chain polymers containing stable radicals. Macromol. Chem. Phys. 1994, 1994, 1017–1030.
54. S. Greve, V. Vill, and W. Friedrichsen. Novel nitronyl nitroxides: synthesis and properties. Z. Naturforsch. 2002, 57b, 677–684.
55. F. D. Saeva, G. A. Reynolds, and L. Kaszczuk. Liquid-crystalline cation-radical charge-transfer systems. J. Am. Chem. Soc. 1982, 1982, 3524–3525.
56. H. Strzelecka, V. Gionis, J. Rivory, and S. Flandrois. Liquid crystalline organic conductors. J. Phys. 1983, 44(C3), 1201–1206.
57. C. V. Yelamaggad, A. S. Achalkumar, D. D. S. Rao, M. Nobusawa, H. Akutsu, J. Yamada, and S. Nakatsuji. The first examples of discotic radicals: columnar mesomorphism in spin-carrying triphenylenes. J. Mater. Chem. 2008, 2008, 3433–3437.
58. S. Castellanos, F. Lopez-Calahorra, E. Brillas, L. Juliá, and D. Velasco. All-organic discotic radical with a spin-carrying rigid-core showing intracolumnar interactions and multifunctional properties. Angew. Chem. Int. Ed. 2009, 2009, 6516–6519.
59. H. G. Aurich. Nitroxides. In: S. Patai and Z. Rappoport,Eds., Nitrones, Nitronates and Nitroxides, John Wiley and Sons, New York, 1989.
60. F. A. Neugebauer. Hydrazidinyl radicals: 1,2,4,5-tetraazapentenyls, verdazyls, and tetrazolinyls. Angew. Chem. Int. Ed. 1973, 1973, 455–464.
61. N. Azuma, K. Ishizu, and K. Mukai. Magnetic-properties of verdazyl free-radicals. VII. π-Bridged verdazyl biradicals with triplet ground-state. J. Chem. Phys. 1974, 1974, 2294–2296.
62. Y. Miura. Synthesis and chemical behaviors of persistent thioaminyl and related radicals. Rev. Heteroatom Chem. 1990, 1990, 211–232.
63. S. Goldschmidt and K. Renn. Bivalent nitrogen: α, α-diphenyl-beta-trinitrophenyl-hydrazyl (IV. Announcement on amine oxidation). Berichte der Deutschen chemischen Gesellschaft 1922, 1922, 628–643.
64. K. Mukai, K. Ueda, K. Ishizu, and Y. Deguchi. ESR studies of the anomalous phase transition in crystalline galvinoxyl radical. J. Chem. Phys. 1982, 1982, 1606–1607.
65. K. Awaga, T. Saugano, and M. Kinoshita. Ferromagnetic intermolecular interactions in a series of organic mixed-crystals of galvinoxyl radical and its precursory closed shell compounds. J. Chem. Phys. 1986, 1986, 2211–2218.
66. O. Armet, J. Veciana, C. Rovira, J. Riera, J. Castaner, J. Molins, J. Rius, C. Miravitlles, S. Olivella, and J. Brichfeus. Inert carbon free-radicals. 8. Polychlorotriphenylmethyl radicals: synthesis, structure, and spin-density distribution. J. Phys. Chem. 1987, 1987, 5608–5616.
67. N. Ikuma, R. Tamura, S. Shimono, Y. Uchida, K. Masaki, J. Yamauchi, Y. Aoki, and H. Nohira. Ferroelectric properties of paramagnetic, all-organic, chiral nitroxyl radical liquid crystals. Adv. Mater. 2006, 2006, 477–480.
68. N. Ikuma, R. Tamura, K. Masaki, Y. Uchida, S. Shimono, J. Yamauchi, Y. Aoki, and H. Nohira. Paramagnetic FLCs containing an organic radical component. Ferroelectrics 2006, 2006, 119–125.
69. Y. Uchida, R. Tamura, N. Ikuma, S. Shimono, J. Yamauchi, Y. Aoki, and H. Nohira. Synthesis and characterization of novel all-organic liquid crystalline radicals. Mol. Cryst. Liq. Cryst. 2007, 2007, 213–221.
70. Y. Uchida, R. Tamura, N. Ikuma, J. Yamauchi, Y. Aoki, and H. Nohira. Synthesis and characterization of novel radical liquid crystals showing ferroelectricity. Ferroelectrics 2008, 2008, 158–169.
71. Y. Uchida, R. Tamura, N. Ikuma, S. Shimono, J. Yamauchi, Y. Shimbo, H. Takezoe, Y. Aoki, and H. Nohira. Magnetic-field-induced molecular alignment in an achiral liquid crystal spin-labeled by a nitroxyl group in the mesogen core. J. Mater. Chem. 2009, 2009, 415–418.
72. C. Kumar, Ed., Magnetic Nanomaterials, Wiley-VCH, Weinheim, 2009.
73. S. T. Lagerwall. Ferroelectric and Antiferroelectric Liquid Crystals, Wiley-VCH, Weinheim, 1999.
74. R. Kogo, F. Araoka, Y. Uchida, R. Tamura, K. Ishikawa, and H. Takezoe. Second harmonic generation in a paramagnetic all-organic chiral smectic liquid crystal. Appl. Phys. Express 2010, 2010, 041701.