Chapter 12
Ferroelectric Colloids in Liquid Crystals
12.1 Introduction
For many years the science of liquid crystals mostly served the needs of liquid crystal display (LCD) industry; vast majority of funds and human resources were directed to development of numerous LCD modes in nematic liquid crystals (LCs). It was the needs of LCD industry that initiated rapid development of surface LC science, deep studies of correlation between the molecular structures and macroscopic properties of nematic LCs. Studies of more complicated LCs phases and composite LC materials to a large extent have also been initiated by numerous attempts to propose competitive alternative to the traditional nematic LCDs (e.g. PDLCs, bistable LCDs, ferroelectric LCDs). By the beginning of the last decade, the LCD industry has reached such a high level that its further progress has become determined mainly by development of the technology of non-LC components (for example, by fabrication of gigantic high-quality glass substrates for the last generation LCDs). This initiated some kind of rebooting of the scientific and mercantile interests of the LC scientific community. There are many sectors of hi-tech industry where LCs have great potentials, such as biotech, telecommunication, and optical processing. The new applications require new materials, sometimes with rather exotic properties, and new technologies. For example, LC materials for telecommunications usually require LCs with strong birefringence but low refractive index, adaptive LC optics needs materials with huge birefringence and low viscosity. Many promising applications of LC for terahertz region are suppressed by a strong absorption of LCs in this region; biotech needs replacement of thermotropic LCs to water-based lyotropic LCs. Tiny and precise patterning of LC alignment over the boundary surfaces becomes crucial for developing new optical elements. All of these new industry needs changed the priority points of LCs science; a number of LCD-related publications steadily decrease in expense of publications on application of LCs in photonics, optical processing, biosensors, and magneto-optics.
One of the directions of the development of the modern LCs science is design and study of numerous LC composite materials. Long-distance orientation interaction in mesophase leads to extremely strong influence of a dispersed material on the mesogenic properties of the LC and vice versa; the LC matrix can effectively arrange the positional and translational ordering of the inclusions in the matrix. Therefore, combination of the orientational ordering and relative translation freedom in LCs with properties of the dispersed materials allows scientists to give unique properties to the composite, which are not inherent to its components. In order to obtain synergetic properties, part of the dispersion material in a LC matrix should not be high. Apparently, Brochard and de Gennes [1] first suggested that doping of a nematic LC with elongated submicron ferromagnetic particles in very low volume fraction (fν ≤ 10−3) should result in drastic increase of the LC sensitivity to a magnetic field. In their picture, the magnetic moments of the particles are aligned by the magnetic field. The coupling between the magnetic particle and the liquid crystal molecule orientations then transfers the magnetic orientational effect onto the underlying liquid crystal matrix. After more than 30 years it was shown that doping of ferroelectric particles at low concentrations to a nematic introduces the ferroelectric properties inherent to the particles [2]. In particular, the nematic becomes sensitive to the sign of the applied electric field. Thus, one can say that the particles in tiny concentration may share their intrinsic properties with the LC matrix. It opens astonishing perspectives of low-concentrated LC nanocolloids for developing unique mesogenic materials and offers an innovative effective means to control precisely the physical properties of liquid crystals.
To realize application potential of the low-concentrated colloids the particles, there should be not only few enough particles but they also should be small enough in order not to disturb the director of a LC. To achieve this, the single particle should be so small that the anchoring parameter ξ = WRpart/K would be much smaller than 1 (W is the anchoring energy of a LC with the surface of the particle, is the elastic constant of a LC, 2Rpart is the characteristic size of the particles). The typical values of the anchoring energy are in the range of 10−4–10−6 J m−2,
. It means that ξ ≤ 1, which corresponds to Rpart ≤ 100 nm. When this condition is met, the particles do not substantially disturb the orientation of the LC director producing a macroscopically uniform alignment. One can say that the director does not “see” the particles, and the colloid appears similar to a pure LC with no readily apparent evidence of dissolved particles.
Despite the director is not disturbed by the nanoparticles, the interaction between them and LC molecules can essentially change the mesogenic properties of a LC. General description of the effect of nanoparticles on LC properties was proposed by Gorkunov and Osipov in the framework of molecular mean-field theory [3]. Effective anchoring potential between a nanoparticle and LC molecules can be written as
(12.1)
where is the long axis of the LC molecule
and
of the axis of the nanoparticles
. The particular expression for the constant
depends on the nature of a LC–nanoparticles interaction (dipole–dipole, van-der-Waals, etc.). The specific form of
determines the effect of the particles on the LC matrix. Even if the particle is isotropic and spherical
, it changes the mesogenic properties of a LC effectively “diluting” it and decreasing the ordering and the clearing temperature Tc:
(12.2)
where Tc,0 is a clearing temperature of the LC host. For the low concentrated colloids this factor decreases Tc by tenth of degrees [4].
If the nanoparticles are anisotropic in shape, they are aligned by the LC matrix and improve the liquid crystal ordering due to the surface anchoring. It leads to the increase of Tc and softening of the first order nematic–isotropic transition. The shape anisotropy factor typically increases also by tenth of degrees [5].
Other types of the anisotropic interactions between nanoparticles and a LC, such as the ones induced by intrinsic ferromagnetism and ferroelectricity of the particles, can lead to additional effect on a LC matrix and essentially change its basic parameters, such as ordering [6, 7], dielectric and conductive [8, 9], magnetic [10, 11], electro-optical [12, 13], and nonlinear optical [14, 15] properties.
When the actual size of the nanoparticles in LC, Rpart becomes smaller than 100 nm, we enter the world of nano-science where the properties of the particles themselves can vary drastically. In sufficiently large colloidal particles, the ferromagnetic and ferroelectric materials form a polydomain structure that usually transforms into a single domain structure when the particles become smaller than 10–20 nm in the case of ferroelectrics [16] and 100–300 nm in the case of ferromagnetics. The further decrease of the size of the single domain ferromagnetics may result in their transition to a single domain superparamagnetic phase, which usually occurs when the particles get smaller than 20 nm [17]. As the size of single ferroelectric particles get smaller than 5 nm, the internal mean field becomes insufficiently high to maintain ferroelectric states, which results in loss of ferroelectricity [16]. The position of plasmon resonance of the noble metal nanoparticles also changes strongly as their size decreases [18]. If we remember about severe aggregation in a mesophase as well, it becomes evident how complex the physics of LC colloids is and how delicate the balance between the size and concentration of the particles in the LC matrix has to be in order to obtain reliable and predictable properties of the suspensions.
As we see, development and study of low-concentrated LC colloids promise us not only new astonishing applications of LCs, but also interesting and fundamental physics. Below we describe the main achievements in physics of low-concentrated LC colloids focusing on the colloids of ferroelectric materials in LCs. Despite the great interest, the detailed description of other types of particles (carbon nanotubes, fullerene dopings, quantum dots, ferromagnetic particles, aerosil, etc.) was left beyond the scope of this chapter.
12.2 Particles Interaction and the Problem of Colloid Stability
There have been no detailed studies of the stability of LC colloids published so far. We found only few brief mentions about the aggregation and sedimentation of the nanoparticles in LCs in the literature [19–22]. At the same time, these processes in thermotropic LC matrixes are very strong. Even if the colloid is visibly stable in the isotropic phase, the transition to the mesophase usually results in formation of visible aggregates, typically in the region of the transition interface. Usually the following attempts to break sedimintated particles and aggregates are not successful: after the refreshment of the dispersion visible aggregates and sedimintated particles appear again. The increased instability is inherent to all kinds of colloidal nanoparticles in LCs regardless the particles' nature (ferroelectric, ferromagnetic, dielectric, semiconductor, metal).
It is evident that the stability problem of LC colloids is directly related to the orientational ordering in a mesophase. The interaction of a nanoparticle with an LC matrix changes the arrangement of LC molecules around the particle disturbing both the order parameter and the director. It increases the free energy of the system, and aggregation of the particles is a mechanism of the compensation of these energy expenses. The trigger of the aggregation is a Brownian motion of the particles. By means of a random walk, the particles are brought at a critical distance lcr, at which the interaction energy is stronger than the thermal energy kBT. As a result, they begin to approach each other and form aggregates [23].
If the particles are so large that the anchoring parameter (it usually corresponds to Rpart = 1–10 μm), the director is strongly disturbed around the particles down to creation of the point of orientational defects nearby [19]. In this case the particles interact as two effective dipoles due to elastic interaction and the critical distance lcr is in the order of tens of microns or less.
For the particles with radius Rpart = 0.1–1 μm, the parameter ξ is usually less than 1. The strong director distortions require too much energy, and the distorted structure transforms into a smooth director deviation around the particle [24]. In this case the particles interact as quadruples, which is several times weaker than the dipoles. As a result, the distance, lcr is in the order of 1 μm.
For the actual “nano”-particles (Rpart ≤ 10–50 nm), and the director does not “see” the particles at all. For this size of the particles, the interaction due the changes of the order parameter near the particles comes to the front [25, 26]. To compensate distortions of the order parameter, the particles begin approaching each other as long as they are at a distance lcr of tens nanometers from each other. Thus, for any size of the particles and any type of interaction between the particles and a LC, the ordering of a mesophase encourages aggregation. Since a probability for the particles to be closer to each other is less than that to be further to each other, a time of the aggregation due to the mesogenic forces for small nanoparticles is much longer for the particles of micron size.
The direct interaction between particles may enhance the aggregation even further. It is clearly demonstrated for the case of carbon nanotubes. These almost one-dimensional objects have the diameters of several or tens of nanometers and the lengths of up to several microns. Strong anisotropy in the combination with great polarisability along the tube axis leads to a strong van-der-Waals interaction, UvdW, between the nanotubes. The numerical calculation [22] has shown that for the radius of the nanotubes Rtube = 50 nm, and their length ltube = 5 μm, the equilibrium spacing of two parallel nanotubes is around 100 nm and their interaction energy UvdW/kBT ≈ 6 × 106. This explains a tendency of a strong aggregation of the nanotubes in LCs. Herewith the anisotropy of LC matrix leads to formation of aggregates elongated to various degrees and predominantly oriented along the director of an LC [20, 22], Figure 12.1a.
Figure 12.1 Photos of the suspensions in optical microscope. (a) Suspension of carbon nanotubes in LC 5CB (fw = 0.025%); scale. (b) Suspension of ferroelectric particles Sn2P2S6 in LC 5CB (fw = 0.1%). (c) Suspension of ferromagnetic particles γ-Fe2O3 in LC 5CB (fw = 0.01%). Courtesy O. Buluy and O. Kurochkin.
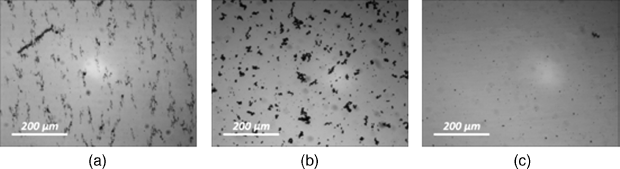
The direct interaction is also important in the case of ferroelectric particles. For two ferroelectric particles, dipoles of which are antiparallel, the electrostatic interaction is
(12.3)
where dpart = PpartVpart is a permanent dipole moment of the particle, P is a polarization (dipole moment per volume unit), Vpart is the particle volume. For typical ferroelectric material BaTiO3, Ppart = 0.26 C/m2 and the particles' size Rpart = 5 nm, the value of Eel is equal to the thermal energy kBT at r = lcr ≈ 350 nm and for r = 100 nm Eel/kBT ≈ 40. Thus, for ferroelectric particles the electrostatic interaction is larger than the interaction due to the ordering distortion. It determines strong aggregation of ferroelectric nanoparticles in a mesophase (Figure 12.1b).
For two ferromagnetic particles with antiparallel dipoles' arrangement the magnetic interaction is
where mpart = μ0 MV, is a magnetization of the material, μ0 MV is a magnetic moment per unit volume. For the typical ferromagnetic material magnetite M = 4.46 × 105 A/m and the particles' size Rpart = 5 nm, the value Emag at r = lcr ≈ 3 nm and for r = 100 nm Emag/kBT ≈ 10−3. It means that the direct magnetic interaction is not crucial for ferromagnetic LC colloids and it is easier to get the stable colloids of such particles rather than of the ferroelectric ones (Figure 12.1c). The same concerns dielectric, semiconductor, and metal nanoparticles interacting mainly due to dipole-induced forces which turn out to be rather weak.
So, for the nanoparticles of the size less than tens of nanometers the aggregation is mainly caused by the direct interaction and the disturbance of the order parameter. In order to decrease the effect of these factors, the particles' surfaces are covered with surfactants. The role of a surfactant is to increase the excluded volume (in other words, to increase the steric repulsion radius) and to “smooth out” the disturbance of the order parameter of the LC around the particle, which is produced due to interaction of LC molecules with the particle' surface.
The most frequently used surfactants are long-chain carboxylic acids, especially an oleic acid (Figure 12.2), molecules of which contain strong polar groups and long hydrocarbon tails that fits well into a LC matrix. The molecules of the oleic acid are attached to the particles surfaces with the polar heads mainly by hydrogen bonds. To cover the particles by the oleic acid, the dispersion of the particles in a solvent (e.g. heptane) is prepared, and the molecules of the acid are spontaneously adsorbed on the particles surfaces with the polar heads. The oleic acid molecules are not too long and do not increase the excluded volume much. At the same time, oleic acid is widely used in ferroelectric and ferromagnetic LC colloids, increasing stability of nanoparticles in LCs compared to the case of uncovered particles. Apparently, the oleic acid molecules, which are well embedded in a LC matrix, soften the disturbance of the order parameter in the particle–LC interface that decreases interparticles interaction.
Figure 12.2 Chemical formula of oleic acid.
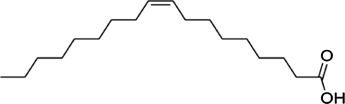
The drawback of the oleic acid as a surfactant is that it is physically absorbed on the particle's surface and some equilibrium fraction of the oleic acid molecules end up in the bulk of a LC matrix decreasing its ordering and clearing temperature . The ratio between the oleic acid molecules on the particles' surfaces and in the bulk can be monitored by IR spectroscopy [27], but the precise control of this ratio is difficult, which is a source of poor reproducibility of the colloid characteristics. Gupta et al. showed [28] that stearic acid gives suspensions that are more stable, but the deposition of material on the particles requires rather tricky treatment. Potentially much better results one can expect from surfactants that are chemically bonded to the surface (chemisorption) by changing the carboxylic function with –SO3H or with phosphorous containing acids [29–32]. In order to optimize interaction of the surfactant with a LC matrix, a mesogenic fragment is attached to the outer end of long flexible hydrocarbon tails chemically bonded to the particle's surface. An example of such surfactant is presented in Figure 12.3 [33]. Application of this surfactant allowed getting stable suspension of elongated ferromagnetic nanoparticles γ-Fe2O3 (diameter, dpart ≈ 15 nm, length, lpart ≈ 100 nm, weight fraction, fw ≈ 5 × 10−3%) in a LC 5CB with no aggregates visible in optical microscope.
Figure 12.3 Chemical formula of the surfactant with the mesogenic group [33].

The dendrite-like surfactant (Figure 12.4) provides a stable suspension with no visible aggregates of even larger elongated magnetic nanoparticles γ-Fe2O3 (dpart =10–25 nm, lpart = 100–150 nm) in a smectic-C LC [34].
Figure 12.4 Chemical formula of the dendrite-like surfactant [34].
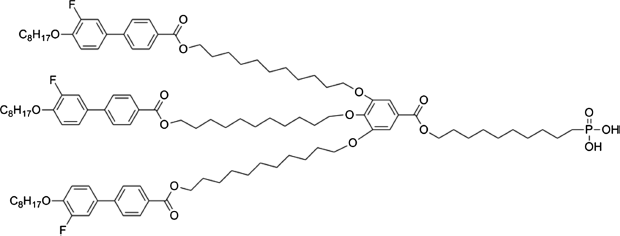
To optimize the interaction between the LC molecules and the surfactant, two-component surfactants are used. One of the components is a long flexible molecule (2–6 nm) that plays an “anti-aggregator” role. The second component consists of short (up to 1 nm), alkyl-containing molecules that cover a part of the particle (undercoating). This arrangement allows LC molecules to penetrate between the long flexible components and to “smooth out” the order parameter variation around the particles. The two-component surfactant depicted in Figure 12.5 [35] has allowed to get a stable suspension of golden nanoparticles (dpart ≈ 1.6 nm) with the weight fraction fw = 1 % in a LC E7 [36]. This suspension did not contain aggregates visible in optical microscope.
Figure 12.5 Gold nanoparticle attached with two-component surfactants [35].
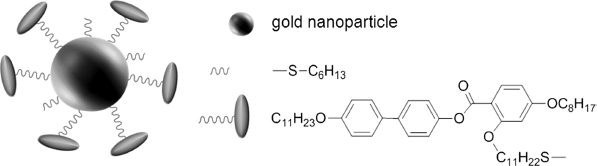
The results of the use of mesogenic surfactants chemically attached to the surface are very promising but the fact that the aggregates were not observed in optical microscope could not serve as a proof of single particles in a LC matrix. The first unambiguous proof that the dispersion of single particles can be produced in LC was obtained for the colloid of quantum dots CdSe/ZnS in a 5CB [37]. The dispersion of quantum dots covered with an organic shell consisting of oleic acid and trioctylphosphine oxide shows clearly visible aggregates with bright yellow luminescence in a fluorescent microscope (Figure 12.6a). In contrast, the quantum dots after site-exchange with mixture of dendron-like surfactant (Figure 12.3) and hexylphosponic acid in ratio 1:4 forms a stable dispersion in 5CB and homogeneously illuminated area is observed in a fluorescent microscope (Figure 12.6b).
Figure 12.6 Fluorescence microscope images of CdSe/ZnS covered with different surfactants; (a) oleic acid based surfactant; (b) dendrite-like surfactant. Courtesy V. Vashchenko.
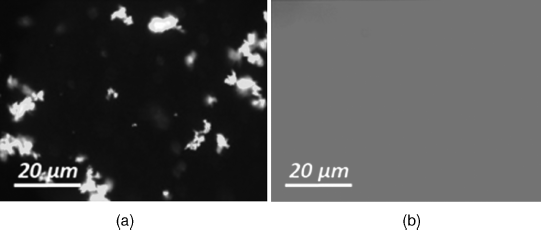
12.3 Preparation of the Ferroelectric Colloids
It is well known that the properties of nanoparticles can drastically differ from the properties of the macroscopic samples and this factor should be taken into account at the each stage of the LC suspensions preparation. It especially concerns ferroelectric particles, which can change or even lose their properties at the nano-scale. In sufficiently large colloidal particles, ferroelectric material is expected to form a polydomain structure. Macroscopic polarization of such particles is very small and working with single domain particles is preferable. The transition to a single domain structure occurs as the size of the particles decreases to approximately 15–20 nm [16]. At further decrease of the particles' size the ferroelectric mean field turns out to be insufficient to maintain a ferroelectric ground state. It usually occurs for the particles smaller than 5 nm. For this reason, in order to get the maximum output of the permanent polarization, the optimal size of the particles in LC colloids should be in the range of 10–100 nm. At the same time, to get the stable colloid with no director disturbance, it is preferable to have the smallest particles possible. This means that the preferable size of the particle should be close to 10 nm. Therefore, only very narrow region of the particles' size can provide efficient influence of the ferroelectric particles. If one also takes into account that the electric charges which always are in a LC bulk can considerably screen the permanent dipole moment of the particles, producing of stable and reliable ferroelectric LC nanocolloids can be a real challenge.
Common techniques of fabrication of nanoparticles by chemical precipitation and spark plasma technique do not work in the case of ferroelectric nanoparticles in LCs, because these techniques usually give either the particles with not-ferroelectric cubic structure or they are too small to have strong ferroelectric properties. Therefore, the primary technique of ferroelectric particles producing is a mechanical grinding of ferroelectric materials [38].
Usually, the ferroelectric material is milled together with a surfactant in a non-ionic liquid carrier. The most popular surfactant is oleic acid and heptanes or ethyl alcohol is taken as the carrier. In some modification of this technique, there is no carrier and only particles with a surfactant are being ground [2].
The final size of the nanoparticles is determined the milling time that in turn, strongly depends on the relative concentration of the components (surfactant, solvent, ferroelectric material), type of the mill (power of the mill, material, and weight of the jar and the ball(s) are important) and temperature of the milling. Depending on the type of the mill and the ferroelectric material, the time to get single domain ferroelectric particles varies from tens of minutes to hundreds of days.
The optimal parameters of the milling also strongly depend on the material being ground. For instance, due to the impact of the balls of planetary ball mill PM200 manufactured by Retsch GmbH the material St2P3S6 is decomposed during the milling and a low powerful mill (e.g. Pulverisette 7 by Fritsch GmbH) should be used for this material. Moreover, the impact of the balls can be so strong that some material from the jar can be dislodged and get incorporated in the ferroelectric powder. This leads to the contamination of the ferroelectric.
All listed factors show how delicate the mechanical grinding technique is. Each combination of the milling machine and the material being ground requires a specific recipe to produce the single domain ferroelectric particles. The optimization of the grinding parameters for a particular material is a long and laborious process, but once it is established the results are very reliable.
Even the optimal grinding parameters do not guarantee the maximum possible polarization of the nanoparticles. Due to the size dispersion, electric charges in the solvent, presence of the particles with the cubic symmetry and other factors only a part of the produced nanoparticles have an essential polarization. This greatly complicates producing the efficient and reliable colloids. The breakthrough in solving this problem was done by Cook et al. [39]. They proposed a technique of harvesting ferroelectric nanoparticles to separate polar and nonpolar particles in the suspension and pick up single domain ferroelectrics particles only. The idea of the technique was to use gradient electric field to selectively harvest ferroelectric nanoparticles with the strongest dipole moments from bulk nanoparticle preparations. The harvesting was performed in a small sealed glass container with the dispersion of ferroelectric particles and surfactant in non-ionic and non-conducting solvent (usually heptanes) (Figure 12.7). There was a thin inner wire electrode at the center of the container and an external radial foil electrode that wrapped around the container. The inner wire electrode was put within a thin-walled sealed glass capillary tube. A high DC potential (typically 10–20 kV in a 2 cm diameter vial) was applied to the inner wire electrode while the outer foil electrode was grounded. When the DC-field was applied, which produced a large field gradient, the harvested nanoparticles with permanent dipoles were accumulated on the inner wire electrode and nonpolar particles without dipole moments were either rejected and accumulated on the outer glass wall or remained within the fluid.
Figure 12.7 The part of the harvesting unit made by G. Cook and D. Evans according to according to Cook et al. [39]. One can see the central wire with the harvested particles on it. Photo of Yu. Reznikov.
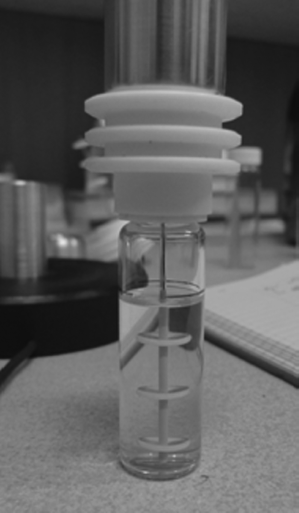
Using the harvesting technique, authors of [39] were able to pick up 9 nm nanoparticles of BaTiO3 and showed that the harvesting essentially enhances the effect of the particles on the characteristics of the LC (decreases the Freedericksz transition voltage and amplifies the photorefraction response). Another important result was that the authors of Ref. [39] were unable to harvest nanoparticles fabricated by direct chemical synthesis, which resulted in nanoparticles' not having dipole moments. At the same time, harvesting can sometimes be successful even when the initial material for the grinding process was produced chemically and had a cubic symmetry. It allowed the authors of Ref. [39] to suggest that the stress and strain in nanoparticles that are produced during the grinding is an important factor in obtaining single ferroelectric domains at the nanometer scale. Moreover, recent experiments of the same group have shown that the ferroelectric properties of small nanoparticles (<10 nm) are enhanced with respect to the large nanoparticle [40].
Our experience has shown that chemically the same ferroelectric materials (e.g. BaTiO3) obtained from different sources or treated differently have an absolutely different harvesting efficiency. Therefore, a prior harvesting is a necessary procedure for the preparation of the reliable ferroelectric LC colloids.
After the grinding the produced nanoparticles need to be transferred to the LC matrix. In order to do this, the suspension of the nanoparticles in a solvent (preferably after the harvesting) is mixed with an LC and carefully dispersed by ultrasonication. Then, the solvent (usually heptanes) is slowly evaporated at slightly elevated temperature and atmosphere pressure. Typically this process lasts around 10 h at 60oC.
It should be noted that even small residuals of the solvent deteriorate the ordering of the LC matrix and mask the effect of the particles. Therefore, the evaporation process should be carefully controlled and monitored, for example, by the precise weighting of the mixture. Special attention should be paid to the solvent evaporation in a case of many-component LC mixtures because this process may cause a change in the matrix composition due to evaporation of low-weight components [7]. The consequent changes of the mesogenic properties of the matrix (average order parameter, , clearing temperature, Tc, dielectric anisotropy, εa, birefringence, na) can be incorrectly interpreted as the effect of nanoparticles. Specifically, evaporation of the heptanes from the mixture of MLC-6609, nanoparticles BaTiO3 and oleic acid at low pressure and elevated temperature led to loss of low-weight components of MLC-6609 mixture that resulted in increase of Tc by several degrees.
It should be underlined that each new combination of the ferroelectric material, surfactant, and LC matrix requires their own fabrication recipe and we described only the principles of the producing of ferroelectric colloids. More detailed information about preparation of some specific colloids are available in the papers [2, 38, 39, 41].
12.4 Orientational Ordering in Ferroelectric Liquid Crystal Colloids
The primary factor that distinguishes ferroelectrics from dielectric and semiconductor materials is a spontaneous electrical polarization. This polarization is a consequence of a the ions shift in the crystal lattice below the Curie temperature TCurie, or an ordering of the microscopic dipoles at T < TCurie. Obviously that it is the permanent dipoles of the ferroelectric nanoparticles that determine the specific properties of the ferroelectric LC colloids. The permanent dipole induces a field in the surrounding isotropic medium.
This field is very strong and is of the same order of magnitude that are the fields dealt with in a nonlinear optics; it is also comparable to the intermolecular fields. For the particles of BaTiO3 with dpart = 10 nm, . The estimation of the ratio of the electrostatic interaction associated with this field to the thermal energy, Upart/kBT gives the number of the order of 104 [42]. Therefore, even if the anisotropic part of the electrostatic interaction is small, one can expect that most of the dipole moments of the particles will be aligned parallel or antiparallel to the local director,
, of a matrix and effective anchoring energy
that describes the coupling between the particles dipole moment and the local director can be considered strong. If the particle is elongated with the dipole moment parallel to the long axis and the LC molecules are parallel to their surfaces (planar alignment conditions), the orientational coupling between the particles and LC is further strengthened and long axis of the elongated particles is also aligned along the director of an LC (Figure 12.8).
Figure 12.8 Schematic illustration of ferroelectric nanoparticles suspended in a liquid crystal. The permanent dipole moments of the nanoparticles have a distribution of orientations coupled with the distribution of orientation of LC molecules.
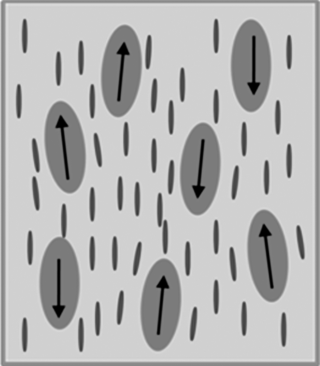
Besides the orientational coupling between the LC matrix and the dipoles, the electric field strongly polarizes the surrounding LC molecules, thereby increasing intermolecular interaction [6]. According to the classic Mayer-Saupe mean field theory, this leads to the increase of the order parameter nearby the particles and, in turn, to the increase of the average order parameter of an LC with the particles,
, and its clearing temperature, Tc. According to Li et al. [6], the shift of Tc does not depend on the size of the particles at the fixed volume fraction fν and is proportional to
:
where Z is nearest neighbour molecules separated by distance lm–m, NLC is the LC molecular concentration, βa is the anisotropy of molecular polarisability. Substitution of the characteristic values in Eq. (12.6) gives the value of ΔTc ≈ (1–10)oC, that is, the effect of the particles should be strong.
With the approach by Li et al. [6], it was implicitly assumed that the dipole moments of all the particles were perfectly aligned in one direction. Lopatina and Selinger [43] considered interaction of the orientational order parameter of the dipoles with the orientational ordering of the liquid crystals. Using Landau theory, which suggested a small order parameter of both a LC and nanoparticles, they showed that it also stabilized the nematic phase and increased Tc. The problem of the application of this theory arises when the order parameter of nanoparticles is not small due to a strong interaction between nanoparticles and liquid crystal molecules. More adequate description of the suspension with interacting order parameters, SLC and Spart was proposed in the other paper of the same authors [44]. The Mayer-Saupe theory that do not limit the value of SLC, was applied. In this case
The estimation according to Eq. (12.7) predicts the shift of Tc ≈ 1°. As one can see, the difference between Eqs (12.4) and (12.5) is that ΔTc scales as in Eq. (12.4) but according to Eq. (12.5) it should scale linearly with εa. This difference arises because in the theory of Li et al. the additional interaction between the molecules is a consequence of the polarization of the neighboring molecules by the electric field
which is proportional to βa. This polarization result in additional intermolecular interaction which scales as
. In the theory of Lopatina and Selinger the direct influence of the nanoparticles' electric field which scales linearly with εa is considered. At the present stage it is difficult to be sure which model is closer to reality but in any case, both approaches suggested in Refs [6] and [44] predict a notable increase of the order parameter and clearing temperature of the colloid.
The rise of and Tc should be accompanied by the corresponding increase of all the parameters of a mesophase that are determined by the LC ordering, such as dielectric anisotropy,
, birefringence,
, Frank constants,
, etc. The systematic experiments carried out with the colloid of ferroelectric nanoparticles Sn2P2S6 in a classic one-component LC pentyl-cianobiphenyl (5CB) [45] confirmed these predictions. In Figure 12.9a the temperature dependencies of the order parameter of 5CB doped with fw ≈ 0.3% of Sn2P2S6 are shown. One can see the evident increase of Tc (ΔTc ≈ 3°) and the order parameter
in the colloid compared to the pure LC 5CB. The measurements of the temperature dependencies
and
showed the corresponding increase of these values.
Figure 12.9 (a) Temperature dependences of the order parameter of the pure 5CB (black line), suspension with the increased Tc (red line), suspension with the decrease Tc (blue line). (b) The same dependences in the reduced temperature coordinates [45].
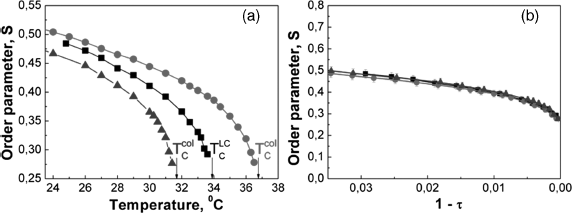
It should be noted that the magnitude and sometimes even the sign of ΔTc and the corresponding changes of and
could vary from sample to sample despite the same producing recipe. The causes of the poor reproducibility of these values are discussed below. The maximum positive shift of Tc was 11° that caused the strong increase of εa and na even at room temperature (Figures 12.10a and b).
Figure 12.10 (a) Temperature dependences of the order parameter of the pure 5CB (black line), suspension with the increased Tc (red line), suspension with the decrease Tc (blue line). (b) The same dependences in the reduced temperature coordinates [45].
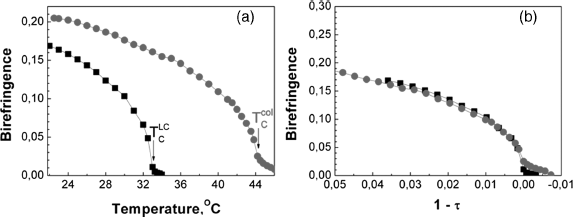
The important point here is that despite the magnitude and even the sign of the changes of the order parameter and clearing temperature vary from sample to sample, in all cases and
plotted as a function of the reduced temperature
fit perfectly (Figure 12.9b). Moreover, the temperature dependencies
and
also fit in the reduced coordinates (Figures 12.10b and 12.11b). It means that all observed changes in the characteristics of the single component LC 5CB can be fully explained by the changes of the order parameter of the LC host due to the presence of the particles. All other possible mechanisms of the changes of the values
and na give only small contribution, if any at all. For instance, it concerns the direct contribution of the dipole moment and polarisability of the particles to
and na. It is also seen from the comparison of the Freedericksz transition voltage in a planar cell filled with a pure 5CB to the one filled with the colloid. The transition voltage for a pure LC is determined by the expression [46]:
Figure 12.11 (a) Temperature dependences of the dielectric constants of the pure 5CB (black line) and the suspension with the increased Tc (red line). (b) The same dependences in the reduced temperature coordinates [45].
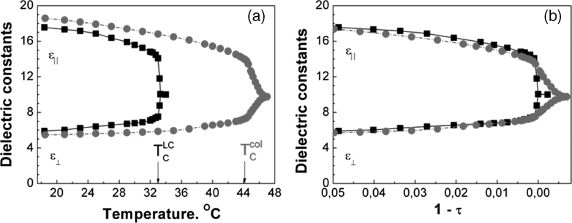
(12.8)
The value , and the elastic constant
[46]. Therefore, if the effect of the particles is reduced to a change of the LC ordering, one can expect a slight increase of the transition voltage
due to increase of the order parameter of the colloid,
. For the experimental data depicted in Figure7a, at room temperature
,
and
are expected. Experimental results confirmed this estimation; it was found that for an AC-field (ν = 1 kHz) the transition voltage ULC = 1.84 ± 0.02 V and Ucol = 1.87 ± 0.02 V.
Thus, one can state that for single-component LC, such as 5CB, the properties of the suspensions are mainly determined by the influence of the particles on the ordering of a LC matrix. Such behavior of the ferroelectric nanoparticles is reminiscent of the behavior of low-molecular weight molecular dopants in nematics. Chen and Luchkhrust as far back as in 1969 concluded that the temperature dependence for a nematic LC with impurities is the universal function of the reduced temperature [47]. It was also shown that the function
did not depend on the chemical structure of the impurities and coincided with the dependence
for the pure LC matrix. Later, Pinkevich et al. [48] showed that this universality follows from the standard Grandjean–Maier–Saupe (GMS) molecular field theory. The sign of the shift of τ is determined by the relationship between the amplitude of inter-molecular interaction of LC molecules, Glc–lc, and the amplitude of interaction “LC molecule—impurity molecule”, Glc–imp. In the case of non-mesogenic impurities, Glc–lc > Glc-–imp, the local order parameter around the impurity is less than the one in a pure LC, and the shift of the clearing temperature, ΔTc < 0. Mesogenic dopants can interact with liquid crystal molecules more strongly than liquid crystal molecules with themselves, Glc–lc < Glc–imp. Therefore, mesogenic impurities can increase the local ordering and increase the clearing temperature.
Exactly the same behavior is observed in the colloid of ferroelectric nanoparticles in 5CB. Therefore, one can suggest that the ferroelectric nano-particles, Sn2P2S6, in LC 5CB can act like molecular dopants, and the change of the order parameter of the LC is the dominant mechanism that determines the difference between the properties of the colloid and pure single component LC.
To understand why such big macroscopic object as ferromagnetic nano-particle, volume of which is 100–1000 times larger than the volume of molecule, can work as a virtual molecular dopant, Reshetnyak considered a ferroelectric LC colloid as LC host with undisturbed order parameter, SLC, with clusters of LC molecules, having order parameter Scl that differs from SLC due to the presence of ferroelectric nano-particles [49]. In this case in the framework of Maier-Saupe model, he obtained universality of the function . The important point is that in this model the universality of the function
is the consequence of the Maier-Saupe formalism that suggests the interaction between LC molecules due to long-range dispersion forces. Therefore, the experiments show that the ferroelectric nano-particles do not change a character of the intermolecular interaction in the LC host, and their influence reduces the enhancement (ΔTc > 0) or depressing (ΔTc < 0) of this interaction, which leads to a change of the host order parameter value.
Finalizing the description of the effect of the ferroelectric nanoparticles on the ordering of LCs, we cannot avoid the question of poor reproducibility of the results in these systems. First of all, one can state that the effect of the increase of the ordering exists unambiguously, and that was independently confirmed by the measurements of the dichroism of dye molecules embedded to the colloid of St2P3S6 in 5CB matrix [45], by the characteristic Raman scattering bands of 5CB molecules in the colloid of BaTiO3 particles in 5CB [41] and by the dichroism of the characteristic functional groups of the nematic matrix components of the suspension of BaTiO3 particles in a MLC-6609 [6]. The question of why in some experiments SLc and Tc decreases, requires additional studies. There are several causes that can mask the effect of the permanent polarization of the particles and lead to a reduction of SLC and Tc.

Figure 12.12 Distribution of the St2P2S6 at the different times of milling. Courtesy O. Kurochkin.
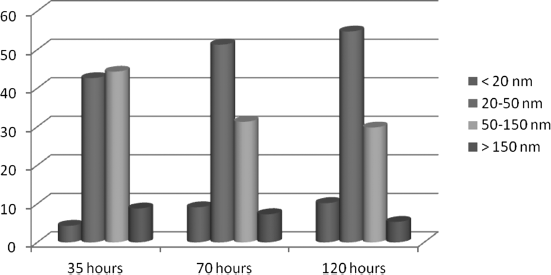
12.5 Dielectric and Reorientational Properties of Ferroelectric LC Colloids
In the previous chapter, we considered the effect of the particles on the properties of a LC matrix due to the mechanism of the orientational coupling enhancement between LC molecules and showed that this mechanism is responsible for the observed properties of a LC 5CB doped with nanoparticles Sn2P2S6. At the same time, we must not forget that the ferroelectrics themselves have the unique dielectric properties. Ferroelectric materials possess spontaneous polarization, their dielectric constants can vary in a wide range ε = 100–10,000. Polarization of ferroelectrics nonlinearly depends on the electric field, it is reversed by the change of the direction of the field and reveals strong hysteresis. In addition, while being in a liquid matrix, ferroelectric nanoparticles can rotate and align according to the sign and the direction of external electric field. All of this must necessarily affect the dielectric properties of an LC and, as a consequence, on the characteristics of reorientational electro-optical effects, that is, determined by dielectric anisotropy of a LC.
There are several experimental evidences of the undoubtful influence of the dielectric properties of the particles on the dielectric and reorientation properties of a LC. First, this is a linear dielectric response of the nematic colloid; when a weak bias electric field is applied to the cell, the director of the colloid is reoriented along the direction of the applied low-frequency (ν = 200 Hz) electric AC-field, , also following the sign of the field [2]. This effect is explained by formation of the polar ordering of the particles' permanent dipoles by the bias field and following collective intact reorientation of the strongly coupled particles and the director with the alternation of the AC-field.
Further, the electrical Freedericksz transition voltage in a DC-field strongly depends on electrical history of the cells with the ferroelectric LC colloid. Cook et al. observed that in a cell with the colloid of BaTiO3 nanoparticle in LC TL205 the voltage of the Freedericksz transition decreased or increased, depending on the polarity of the applied voltage, giving a net 1.6 V Freedericksz threshold asymmetry [50]. This polarization hysteresis indicates that the cell behaves as a ferroelectric material that is, explained in line with [2] by orientation of the particles' dipole moments in a DC-field according to the sign of the field. The interesting fact is that in the experiments of Cook et al. the cell “remembered” the sign of the applied field, sometimes even after overheating of the cell above clearing point. It means that once aligned, the dipole moments may keep their alignment for rather long time, unlike in the case described by Reznikov et al. [2], where the polarization of the cell disappeared during few milliseconds after the bias electric field was switched off.
One more evident contribution of the ferroelectricity to the dielectric properties of the colloids is repeatedly observed strong increase (by several times) of the LCs dielectric anisotropy and birefringence after doping it with ferroelectric particles [2, 28, 41, 51, 52]. In some experiments, this increase was observed despite decrease of Tc in the colloid and cannot be explained by the increase of the LC matrix order parameter [28].
The most developed theory of dielectric properties of ferroelectric LC suspension was recently published by Shelestiuk et al. [42]. The authors generalized the Maxwell-Garnet approach considering anisotropic in shape and dielectric polarisability nanoparticles in a dielectrically anisotropic LC matrix. The particles possess a permanent dipole moment, a strong orientational coupling between the particles and LC is suggested and no interaction between the particles is assumed.
One of the conclusions of the classic Maxwell-Garnet theory is that adding dielectric particles with high dielectric permittivity to a dielectric matrix with much smaller permittivity,
does not lead to a notable increase of the total effective dielectric permittivity of the suspension. The situation is different in the case of ferroelectric particles that possess permanent dipoles, however. Application of even a weak electric field breaks central symmetry of the dipoles' orientation and mean particle permanent polarization in the LC matrix becomes
(12.9)
where and
are the fractions of the particles aligned, respectively, parallel and antiparallel to the local director
. The fractions
and
are described by the Boltzmann distribution in electric field
and lead to the mean value of
, which contributes to the expressions for effective permittivities of the suspension. The analytical, although rather complicated, expressions of
and
can be found in the article [42]. They show that the presence of the permanent dipole moment does increase the value of
and accordingly, the dielectric anisotropy
. Unfortunately, the uncertainty of many experimental parameters (P, Vpart, fν, etc.) makes the quantitative comparison of the calculations with the experimental data difficult at this stage.
The effect of the ferroelectric particles on the dielectric properties of a LC is closely related to repeatedly observed decrease of the Freedericksz transitions voltages in AC-field [2, 28, 41]. Typically, the transition voltage UFr drops by 1.3–2.5 times. These values are too high to be explained by possible disordering of the LC matrix, which results in slight decrease of . The problem about the Freedericksz transitions in the ferroelectric LC suspension was consistently resolved in [42]. The important result of this work is that the effective permittivity as it appears in the expression for free energy of the colloid in a cell does not coincide with the expressions for
and
which are obtained in the frame of generalized Maxwell-Garnett picture. Therefore, the formula for the Freedericksz transitions voltage is given by
(12.10)
where . Since
, the Freedericksz transition voltage decreases in the suspension. The variance between
and
can be serious, and difference in the calculations of the Freedericksz transition voltage reduction is essential.
It should be noted that the experimental data described in this chapter were obtained for multi-component LC mixtures and this circumstance was not taken into account in the theories of dielectric properties and Freedericksz transition of ferroelectric colloids. At the same time, the presence of different components with different molecular masses and dipole moments may seriously affect the final properties of the suspensions. Indeed, an electric field decreases quickly (as r−3) with a distance from the particles. Therefore, the local electrical field is very inhomogeneous in a LC, even if it is partially compensated by free charges which are always there in an LC. Motion of the polar molecules with various dipole moments and various molecular weights in the gradient of the local electric field, as well as various different adsorption affinities of various mixtures' molecular components on the particle's surface can lead to a spatial redistribution of the individual components of the LC matrix. Obviously, the resulting micro/nano-separation of the mixture should affect the macroscopic properties of the colloid. Micro-separation probably provides additional contribution to the changes of the dielectric and reorientation properties in the ferroelectric colloids based on many-component LCs and masks the effect of the orientation amplification, clearly observed in a single-component matrix 5CB.
The effect of the ferroelectric particles on the dielectric and reorientation properties of ferroelectric LC is of special interest because interaction between the permanent polarization of the particles and macroscopic polarization of the LC can be expected. There are only few papers on this topic [54, 55] till now, but they point to a strong effect of the particles. Liang et al. [54] reported on almost doubling of the spontaneous polarization, enhancement of dielectric properties and faster response time in a ferroelectric LC CS1024 (Chisso) doped with BaTiO3 (~30 nm size) in a small concentration. Unlike them, Mikułko et al. [55] observed only slightly lower spontaneous polarization and lower relative dielectric permittivity for the nanocomposite of BaTiO3 (~30 nm size) in another ferroelectric LC LAHS9. At the same time, as in the work of Liang et al., faster response time of the nanocomposite was reported.
12.6 Conclusions
The science of LC ferroelectric colloids is very young and many of its technological and scientific problems are yet to be solved, many issues are under hot discussions and the general description of these materials is far from completion. Nevertheless, summarizing the knowledge obtained since the first publication on the properties of ferroelectric LC colloids, one can list the following main results:
- Strong permanent polarization of the ferroelectric nanoparticles results in unique properties of the LCs doped with these nanoparticles, such as sensitivity to the sign of the electric field, enhanced dielectric anisotropy, and birefringence of nematic LCs.
- There are two main mechanisms of the particles' effect: the increase of the orientation coupling between LC molecules and the direct contribution of the permanent polarization of the particles to the dielectric properties of the LC mixture. The latter is the primary factor in the case of multi-component LC mixtures and the enhancement of the orientation coupling dominates in a single-component LC 5CB. In the last case, the ferroelectric nanoparticles can be considered as effective molecular impurities with giant dipole moments.
- Only narrow range of the particles' sizes, approximately from 5–8 to 10–20 nm, results in providing effective influence of the particles. Together with screening of the permanent polarization by free electric charges, it makes it still difficult to produce reliable colloids. The harvesting of the ferroelectric nanoparticles helps to improve the reliability and efficiency of the particles impact.
These basic results established a solid platform for the following fundamental studies and application of ferroelectric LC colloids. We believe that after development of highly reliable methods of the producing stable ferroelectric LC colloids, they will offer an innovative simple and effective means to control precisely the physical properties of liquid crystalline materials and find its important place among LC materials for electro-optical, nonlinear optical, and telecommunication LC devices.
Acknowledgments
I am grateful to my close collaborators, D. Evans, A. Glushchenko, V. Reshetnyak, T. Sluckin, J. West, and their teams for long-term collaboration in the field of LC colloids, and for numerous useful discussions which have helped us to agree on a joint view of ferroelectric LC colloid physics. I also acknowledge the help and advice from O. Buchnev, O. Buluy E. O. Kurochkin, B. Lev, L. Lopatina, A, Morozovskaya, M. Reznikov, V. Zadorozhnyi, and V. Vashchenko. I thank Ch. Rosenblatt for reviewing the manuscript and the valued advice.
1. F. Brochard and P. G. de Gennes. Theory of magnetic suspensions in liquid crystals. J. de Phys. 1970, 31, 691–706.
2. Yu. Reznikov, O. Buchnev, O. Tereshchenko, V. Reshetnyak, A. Glushchenko, and J. West. Ferroelectric nematic suspension. Appl. Phys. Lett. 2003, 82, 1917.
3. M. Gorkunov and M. Osipov. Mean-field theory of a nematic liquid crystal doped with anisotropic nanoparticles. Soft Matter 2011, 7, 4348–4356.
4. F. Haraguchi, K. Inoue, N. Toshima, S. Kobayashi, and K. Takatoh. Reduction of the threshold voltages of nematic liquid crystal electrooptical devices by doping inorganic nanoparticles. Jpn. J. Appl. Phys. 2007, 46, L796–L797.
5. H. Duran, B. Gazdecki, A. Yamashita, and T. Kyi. Effect of carbon nanotubes on phase transition of nematic liquid crystals. Liq. Cryst. 2005, 32, 815–821.
6. F. Li, O. Buchnev, C. Cheon, A. Glushchenko, V. Reshetnyak, Yu. Reznikov, T. Sluckin, and J. West. Orientational coupling amplification in ferroelectric nematic colloids. Phys. Rev. Lett. 2006, 97, 147801/ 1–4.
7. F. Li, O. Buchnev, C. Cheon, A. Glushchenko, V. Reshetnyak, Yu. Reznikov, T. Sluckin, and J. West. Erratum: Orientational coupling amplification in ferroelectric nematic colloids. Phys. Rev. Lett. 2006, 97, 147801/1-4. Phys. Rev. Lett. 99, 219901.
8. R. Basu and G. S. Iannacchione. Nematic anchoring on carbon nanotubes. Appl. Phys. Lett. 2009, 95, 173113/ 1–3.
9. I. Dierking, G. Scalia, and P. Morales. Liquid crystal–carbon nanotube dispersions. J. Appl. Phys. 2005, 97, 044309/ 1–5.
10. P. Kopanský, N. Tomašovi
ová, M. Koneracká, V. Závišová, M. Timko, A. Džarová, A. Šprincová, N. Éber, K. Fodor-Csorba, T. Tóth-Katona, A. Vajda, and J. Jadzyn. Structural changes in the 6CHBT liquid crystal doped with spherical, rodlike, and chainlike magnetic particles. Phys. Rev. E 2008, 78, 011702/ 1–5.
11. N. Podoliak, O. Buchnev, O. Buluy, G. D'Alessandro, M. Kaczmarek, Yu. Reznikov, and T. J. Sluckin. Macroscopic optical effects in low concentration ferronematics. Soft Matter 2011, 7, 4742–4749.
12. O. Kurochkin, O. Buchnev, A. Iljin, S. K. Park, S. B. Kwon, O. Grabar, and Yu. Reznikov. A colloid of ferroelectric nanoparticles in a cholesteric liquid crystal. J. Opt. A: Pure Appl. Opt. 2009, 11, 024003/ 1–5.
13. M. Kaczmarek, O. Buchnev, I. Nandhakumar. Ferroelectric nanoparticles in low refractive index liquid crystals for strong electro-optic response. Appl. Phys. Lett. 2008, 92, 103307/ 1–3.
14. O. Buchnev, A. Dyadyusha, M. Kaczmarek, V. Reshetnyak, and Yu. Reznikov. Enhanced two-beam coupling in colloids of ferroelectric nanoparticles in liquid crystals. J. Opt. Soc. Am. B 2007, 24, 1512–1516.
15. G. Cook, A. V. Glushchenko, V. Reshetnyak, A. T. Griffith, M. A. Saleh, and D. R. Evans. Nanoparticle doped organic-inorganic hybrid photorefractives. Opt. Express 2008, 16, 4015–4022.
16. E. Erdem, H.-C. Semmelhack, R. Bottcher, H. Rumpf, J. Banys, A. Matthes, H.-J. Glase, D. Hirsch, and E. Hartmann. Study of the tetragonal-to-cubic phase transition in PbTiO3 nanopowders. J. Phys.: Condens. Matter 2006, 18, 3861–3874.
17. D. S. Mathew and R.-S. Juang. An overview of the structure and magnetism of spinel ferrite nanoparticles and their synthesis in microemulsions. Chem. Eng. J. 2007, 129, 51–65.
18. S. Y. Park and D. Stroud. Splitting of surface plasmon frequencies of metal particles in a nematic liquid crystal. Appl. Phys. Lett. 2004, 85, 2920–2922.
19. H. Stark. Physics of colloidal dispersions in nematic liquid crystals. Phys. Rep. 2001, 351, 387–474.
20. E. Ouskova, O. Buluy, C. Blanc, H. Dietsch, and A. Mertel. Enhanced magneto-optical properties of suspensions of spindle type mono-dispersed hematite nano-particles in liquid crystal. Mol. Cryst. Liq. Cryst. 2010, 525, 104–111.
21. V. V. Ponevchinsky, A. I. Goncharuk, V. I. Vasil'ev, N. I. Lebovka, and M. S. Soskin. JETP Lett. 2010, 91, 241–244.
22. O. Buluy, S. Nepijko, V. Reshetnyak, E. Ouskova, V. Zadorozhnii, A. Leonhardt, M. Ritschel, G. Schönhense, and Yu. Reznikov. Magnetic sensitivity of a dispersion of aggregated ferromagnetic carbon nanotubes in liquid crystals. Soft Matter 2011, 7, 644–649.
23. S. Chandtasekhar. Stochastic problems in physics and astronomy. Rev. Mod. Phys. 1943, 15, 1–89.
24. O. V. Kuksenok, R. W. Ruhwandl, S. V. Shiyanovskii, and E. M. Terentjev. Director structure around a colloid particle suspended in a nematic liquid crystal. Phys. Rev. E 1996, 54, 5198–5203.
25. P. Galatola and J.-B. Fournier. Nematic-wetted colloids in the isotropic phase: Pairwise interaction, biaxiality, and defects. Phys. Rev. Lett. 2001, 86, 3915–3918.
26. S. Chernyshuk, B. Lev, and H. Yokoyama. Paranematic interaction between nanoparticles of ordinary shape. Phys. Rev. E 2005, 71, 062701/ 1–4.
27. H. Atkuri, K. Zhang, and J. West. Fabrication of paraelectric nanocolloidal liquid crystals. Mol. Cryst. Liq. Cryst. 2009, 508, 183–190.
28. M. Gupta, I. Satpathy, A. Roy, and R. Pratibha. Nanoparticle induced director distortion and disorder in liquid crystal-nanoparticle dispersions. J. Colloid Interf. Sci. 2010, 352, 292–298.
29. A. Szilágyi, G. Fetter, and M. Zrínyi. Thermotropic behaviour of the complex liquid crystal system containing 8CB [4-cyano-4'-(n-octylbiphenyl)] and organic ferrofluid. J. Therm. Anal. Calorim. 2005, 82, 525–530.
30. A. B. Bourlinos, A. Bakandritsos, V. Georgakilas, and D. Petridis. Surface modification of ultrafine magnetic iron oxide particles. Chem. Mater. 2002, 14, 3226–3228.
31. T. Fried, G. Shemer, G. Markovich. Ordered two-dimensional arrays of ferrite nanoparticles. Adv. Mater. 2001, 13, 1158.
32. C. Yee, G. Kataby, A. Ulman, T. Prozorov, H. White, A. King, M. Rafailovich, J. Sokolov, and A. Gedanken. Self-assembled monolayers of alkanesulfonic and phosphonic acids on amorphous iron oxide nanoparticles. Langmuir 1999, 15, 7111–7115.
33. M. Kolosov, A. Krivoshey, M. Prodanov, O. Buluy, Yu. Reznikov, O. Semenenko, J. Goodby, and V. Vashchenko. Dispersion of magnetic nano rods in nematic liquid crystals. 23rd International Liquid Crystal Conference 2010, July 11–16, 2010, Krakow, Poland, Abstract Book.
34. M. Prodanov, A. Fedoryako, M. Kolosov, A. Krivoshey, J. Goodby, V. Vashchenko, E. Pozhidaev, and S. Gamzaeva. Rotational viscosity of dispersion of magnetic nano particles in ferroelectric liquid crystals. 23rd International Liquid Crystal Conference, July 11-16 2010, Krakow, Poland, Abstract Book, p. 742.
35. L. Cseh and G. H. Mehl. The design and the investigation of room temperature thermotropic nematic gold nanoparticles. J. Am. Chem. Soc. 2006, 128, 13377–133786.
36. E. Ouskova, D. Lysenko, S. Ksondzyk, L. Cseh, G. H. Mehl, V. Reshentnyak, and Yu. Reznikov. Strong cubic optical nonlinearity of gold nanoparticles suspension in nematic liquid crystal. Mol. Cryst. Liq. Cryst. 2011, 545, 123–132.
37. M. Prodanov, N. Pogorelova, A. Kurochkin, A. Kryshtal, A. Krivoshey, Yu. Reznikov, J. Goodby, and V. Vashchenko. Dispersion of quantum dots in nematic liquid Crystals. 6th International Chemistry Conference Toulouse-Kiev, May 30–June 1, 2011, Abstrats Book.
38. H. Atkuri, G. Cook, D. R. Evans, C.-I. Cheon, A. Glushchenko, V. Reshetnyak, J. West, and K. Zhang. Preparation of ferroelectric nanoparticles for their use in liquid crystalline colloids. J. Opt. A: Pure Appl. Opt. 2009, 11, 024006/ 1–5.
39. G. Cook, J. L. Barnes, S. A. Basun, D. R. Evans, R. F. Ziolo, A. Ponce, V. Yu. Reshetnyak, A. Glushchenko, and P. P. Baneerje. Harvesting single ferroelectric domain stressed nanoparticles for optical and ferroic applications. J. Appl. Phys. 2010, 108, 064309/ 1–5.
40. D. R. Evans, S. A. Basun, G. Cook, I. P. Pinkevych, and V. Yu. Reshetnyak. Electric field interactions and aggregation dynamics of ferroelectric nanoparticles in isotropic fluid suspensions. Phys. Rev. B 2011, 84, 174111/1–9.
41. J.-F. Blach, S. Saitzek, C. Legrand, L. Dupont, J.-F. Henninot, and M. Warnghem. BaTiO3 ferroelectric nanoparticles dispersed in 5CB nematic liquid crystal: Synthesis and electro-optical characterization. J. Appl. Phys. 2010, 107, 074102/ 1–7.
42. S. Shelestiuk, V. Reshetnyak, and T. Sluckin. Fredericks transition in ferroelectric liquid-crystal nanosuspensions. Phys.Rev. E 2011, 83, 041705/ 1–13.
43. L. M. Lopatina and J. V. Selinger. Theory of ferroelectric nanoparticles in nematic liquid crystals. Phys. Rev. Lett. 2009, 102, 197802–197804.
44. L. M. Lopatina and J. V. Selinger. Maier-Saupe-type theory of ferroelectric nanoparticles in nematic liquid crystals. Phys. Rev. E. 2011, 84, 141703/1-7.
45. O. Kurochkin, H. Atkuri, O. Buchnev, A. Glushchenko, O. Grabar, R. Karapinar, V. Reshetnyak, J. West, and Y. Reznikov. Nano-colloids of Sn2P2S6 in nematic liquid Crystal. Cond. Matt. Phys. 2010, 13, 33701/ 1–9.
46. P. G. de Gennes and J. Prost. The Physics of Liquid Crystals. Oxford University Press, New York, 1994.
47. D. Chen and G. Luchkhrust. Electron resonance study of the perturbation of the order in a nematic mesophase by a second component. Trans. Faraday Soc. 1969, 65, 656–664.
48. I. P. Pinkevich, Yu. Reznikov, V. Y. Reshetnyak, and O. V. Yaroshchuk. Conformational optical nonlinearity of nematic liquid crystals. Int. J. Nonlinear Opt. Phys. 1992, 1, 447–472.
49. Private communication
50. G. Cook, V. Reshetnyak, R. F. Ziolo, S. A. Basun, P. P. Banerjee, D. R. Evans. Asymmetric Freedericksz transitions from symmetric liquid crystal cells doped with harvested ferroelectric nanoparticles. Opt. Express 2010, 18, 17339–17345.
51. M. Kaczmarek, O. Buchnev, and I. Nandhakumar. Ferroelectric nanoparticles in low refractive index liquid crystals for strong electro-optic response. Appl. Phys. Lett. 2008, 92, 103307/ 1–3.
52. O. Kurochkin, O. Buchnev, A. Iljin, S. K. Park, S. B. Kwon, O. Grabar, and Yu. Reznikov. A colloidofferroelectricnanoparticlesin a cholestericliquidcrystal. J. Opt. A: Pure Appl. Opt. 2009, 11, 024003/ 1–5.
53. O. Buchnev, A. Dyadyusha, M. Kaczmarek, V. Reshetnyak, Yu. Reznikov. Enhanced two-beam coupling in colloids of ferroelectric nanoparticles in liquid crystals. J. Opt. Soc. Am. B 2007, 24, 1512–1516.
54. H.-H. Liang, Y.-Z. Xiao, F.-J. Hsh, C.-C. Wu, and J.-Y. Lee. Enhancing the electro-optical properties of ferroelectric liquid crystals by doping ferroelectric nanoparticles. Liq. Cryst. 2010, 37, 255–261.
55. A. Mikułko, P. Arora, A. Glushchenko, A. Lapanik, and W. Haase. Complementary studies of BaTiO3 nanoparticles suspended in a ferroelectric liquid-crystalline mixture. Europhys. Lett. 2009, 87,27009/ 1–4.