Chapter 10
Liquid Crystals of Carbon Nanotubes and Carbon Nanotubes in Liquid Crystals
10.1 Introduction
The combination of liquid crystals (LCs) and carbon nanotubes (CNTs) occupies a special place among the uses of liquid crystals in non-display applications because of the related intriguing scientific issues but also for the very appealing applications. Many articles have been published on different aspects of the topic and a few review articles have appeared [1–7].
Nanoparticles have attracted general attention and carbon nanotubes have come out from the mass for their peculiar and outstanding properties making them one of the most interesting materials of the century. LCs and CNTs seem to belong to two different worlds but they share more similitarities than expected. They are both anisotropic materials and as for LCs the macroscopic use of the nanotube properties relies on a macroscopic control of the tube alignment. This need brings these two worlds together but the interest of their combination goes well beyond.
The topic combines very different research fields bringing along their own specific issues and terminology. It is unavoidable in the early time of the meeting of these two worlds to find now and then in articles imprecise language or terminology from scientists from one community on the part related to the other community. However, this cross-fertilizing process brings new knowledge and applications creating a new research area of great breadth and with its own identity.
Let us first of all introduce carbon nanotubes: they are seamless cylinders of carbon atoms in a honey-comb arrangement. They can be visualized as formed by rolling up a graphene sheet. These tubes possess extremely high aspect ratio with diameters of the order of nanometers and variable length ranging from micrometer up to centimeters. If we consider a moderate length nanotube, let us say 1 micrometer long and with 1 nm diameter, its aspect ratio would correspond in our length-scale to an object with diameter of 1 m and length of 1 km! It is quite impressive to see that an object of such an aspect ratio is in fact stable. To be so it must possess superior mechanical properties.
The article by Ijima [8] in the early 90s boosted an enormous interest toward this nanoscale allotrope of carbon. The extreme aspect ratio makes CNTs quasi-one-dimensional objects, very interesting for basic science. Carbon nanotubes have also displayed exceptional properties [9] that together with their reduced dimensions make them very attractive for innovative applications. They can consist of a single wall, the single-wall CNTs (SWCNTs) or be composed of several concentric layers, a sort of Russian doll, the multi-wall CNTs (MWCNTs). In Figure 10.1 transmission electron microscopy (TEM) images of carbon nanotubes with one wall, single-wall (SWCNTs) on the left, and a MWCNT, on the right are reported. Because of the multiple walls that increase the diameter, MWCNTs typically have a lower aspect ratio than the SWCNTs. It is worth to point out that the progress in the synthesis of CNTs has allowed the realization of samples containing nanotubes with mainly two walls, thus introducing the concept of double-wall CNTs (DWCNTs).
Figure 10.1 Images of CNTs by transmission electron microscopy (TEM). On the left an example of single-wall CNTs, produced through laser ablation by B. Hornbostel and imaged by J. Cech. On the right an example of MWCNTs, produced by arc-discharge by M. Haluska, imaged by J. Cech.
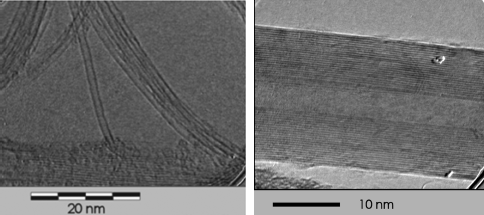
Thanks to the graphitic structure CNTs have extraordinary mechanical properties. They are resilient against stresses with exceptional density-normalized Young's modulus and tensile strength. In SWCNTs those were found some 20 and 60 times, respectively, higher than those of steel [10]. According to the way the graphene layer is “rolled up” the nanotubes have different electronic properties. The identification of the type of nanotube is made via a couple of integer numbers usually indicated with the letters n and m respectively, cf. Figure 10.2. These are the coefficients in the base of the graphene unit vectors, defining the C vector that indicates how the CNT is rolled up. Since there are many different ways or directions of rolling up graphene into a tube, nanotubes with many different characteristics are present in ordinary samples. The nanotubes with n = m are called as armchair from the profile of the arrangement of the carbon atoms on the tube circumference, perpendicular to the axis, while those with m = 0, for a similar reason, are called as zig–zag. These types based on their wall structures are not chiral. Among these two extreme structures a multitude of other arrangements can be obtained by rolling up at intermediate angles generating tubes that are instead chiral. All these intermediate forms have their own (n, m) indices and thus their own properties. In general, CNTs can be metallic or semiconducting, the two categories appearing at a ratio of 1:2, with the width of the band-gap of the semiconducting nanotubes proportional to the inverse of their diameter. The electrical conducting properties of CNTs are highly interesting. They exhibit an electron mobility of 100,000 cm2/Vs, while the mobility in silicon is about 450 cm2/Vs. They can carry a current density in the order of 1010 A/cm, orders of magnitude higher than copper with 106 A/cm2. If the nanotubes have no defects the transport is ballistic, that means without scattering. The thermal conductivity is also remarkably high. In MWCNTs it has been measured to be more than 3 kW/mK [10], greater than that of natural diamond.
Figure 10.2 A single-wall carbon nanotube (SWCNT) can be viewed as a graphene sheet rolled up into a tube. Depending on the roll-up direction (n, m) the tube can be achiral (5.5 and 9.0 for examples, the former giving the armchair tube on the right) or chiral (any direction between the achiral ones allowing closure into a seamless tube, for example the 8.2).
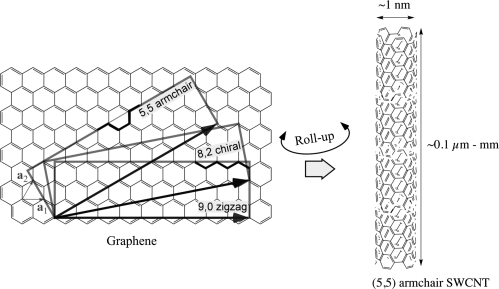
Because of their extraordinary properties many applications have been envisaged. The mechanical properties are attractive in composites or for realizing extra strong fibers. Metallic CNTs are attractive as nanowires in electronic circuits while the semiconducting can be used as transistors. Their use as heat sink in electronic circuits is also proposed together with the use as filler for improving the thermal properties of materials. Other potential uses are tips for AFM, thanks to their extreme aspect ratio and the ultra-small diameter, as actuators, as drug delivery system, others based on their field emission properties and many more. In fact their excellent mechanical, electrical, and thermal properties together with their size make them ideal candidates in nanotechnology and for the realization of novel materials.
Despite the exceptional properties some issues need to be solved for their successful implementation in devices and materials. The presence of impurities and of defected tubes is a very important issue. Also there can be tubes not perfectly straight but bent, kinked, or branched. Defects in the tubes not only worsen their electrical, thermal, and mechanical properties but also influence their order in liquid crystals. As produced CNT samples contain, beside nanotubes, metal catalysts (if used for the CNT growth), amorphous carbon, or graphitic particles. Their presence can affect the properties of composites or the formation of LC phases. Caution must be taken about the presence of these by-products when using as-produced samples. Methods have been developed to purify CNTs and it is possible to purchase purified tubes of different grade or doing it in your own lab. Assessing the purity is not a trivial task and requires a combination of techniques but it is of key importance since the final performance and properties can be strongly affected by the presence of by-products. Ordinary samples contain nanotubes with different properties, different diameters, and also different length. This is a serious problem for carbon nanotube research because it brings a lack of control of the desired properties. The attempts to control the CNT type during growth, in order to have monodispersity and thus CNTs with specific properties, have so far not been successful. More promising are post-growth methods aimed to fractionate samples according to the tube length and diameter.
The CNT properties are excellent but mainly so along the nanotube axis, the extreme anisotropic shape of CNTs bringing strong anisotropy of their properties. The consequence is that the control of the CNT orientation is of great importance for the successful application of CNTs in devices or for the realization of new materials and for improving their performance [11–18]. The control of CNT alignment is thus an important issue and different approaches have been developed so far. Several review articles on the topic can be found in the literature, among them [19, 20]. The alignment methods can be divided into two major groups. The first is based on the realization of CNTs aligned during growth producing vertically aligned tubes referred as “forests” or CNTs planarly aligned on the substrate [21–24]. However, this approach has the disadvantage that CNTs with desired properties, degree of purification or sorted according to the size using the new wet-processing procedures cannot be chosen.
Diverse approaches have been used for obtaining post-growth alignment like application of electric, magnetic fields, mechanical stretching, or other types of directional forces [25–35]. Post-growth alignment methods are preferable to aligned growth because they can profit of the advances in purification and fractionation offering a final material with improved quality. The above mentioned methods of post-growth alignment are often bringing unsatisfactory results or being awkward or not applicable for all CNT types. An easy, versatile and general way of aligning CNTs is still lacking. In the quest of control of the orientation liquid crystal phases or liquid crystalline materials can play a crucial role. The liquid crystal route can be an effective and innovative alternative for manipulating and controlling the organization of the nanotubes.
Carbon nanotubes meet LCs also in other fields. CNTs can form transparent and conductive networks that have been proposed as replacement for ITO, for example in LCDs. They offer the advantage of better mechanical performance and flexibility making them compatible with flexible substrates. On the other hand, they were also used as alignment layer for LCs as first shown by Samulski and co-workers [36]. Carbon nanotubes have also been used as dopant for liquid crystals for enhancing the display related properties [37–46]. Although these topics are interesting and constitute another meeting point with liquid crystal science, they are related to display applications thus their treatment is out of the scope of this book.
In this chapter, the dispersion of CNTs will be discussed due to its key importance in any use of CNTs, giving also space to specific LC related issues. Then the LC phases formed by CNTs will be discussed followed by a short treatment of inclusions of CNTs in low-molar mass thermotropics, then CNTs in lyotropics and finally in polymeric systems. The vast majority of the works in the field have been of experimental nature with a few exceptions of theoretical articles [47–55].
10.2 Dispersion of Carbon Nanotubes
One of the greatest challenges in any work on carbon nanotube suspensions is to efficiently separate the tubes and to keep them separated over long times within the intended host. Since carbon nanotubes are particles consisting entirely of carbon in an aromatic arrangement with an exceptional specific surface area they tend to aggregate very strongly due to very strong van der Waals interactions between adjacent tubes. There are extremely few organic solvents that can be considered useful as carbon nanotube hosts, although acceptable performance is provided by N-methylpyrrolidone (NMP) [56]. More recently, even better results were found with cyclohexyl-pyrrolidinone (CHP) [57]. The most common approach is otherwise to counteract nanotube aggregation by taking advantage of the hydrophobic effect, coating the nanotubes with low-molar mass surfactants [58] or amphiphilic polymers [59, 60] and use water as the dispersion medium. The amphiphiles adsorb onto the hydrophobic nanotube surface with their non-polar components, directing their polar groups outwards toward the aqueous host phase. This first of all renders the 'effective' nanotube surface, as experienced by the world outside the wrapped CNT, hydrophilic, second, the hydrophilic surfactant head groups or polymer chains introduce a repulsive force between the nanotubes, either of electrostatic type (for ionic head groups) or of entropic type (for flexible chains that get entangled in case of close nanotube contact, with a consequent entropy penalty), cf. Figure 10.3.
Figure 10.3 Schematic illustrations of how carbon nanotubes (black rods) are kept dispersed by adsorbed ionic (left) or nonionic (right) surfactants.
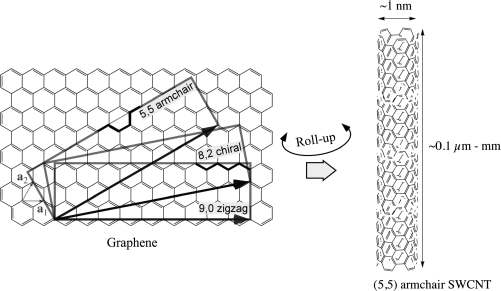
When the dispersion medium is a liquid crystal the amphiphile-based approach is useful for lyotropic liquid crystal hosts [61–73]. A potential problem is however that the large number of micelles present in a surfactant-based lyotropic liquid crystal phase can act as ‘depletants’, that is they may induce aggregation of the nanotubes by means of depletion attraction [74]. As the nanotube-free micelles are repelled from those containing nanotubes, the particles and their surroundings constitute excluded volumes (depleted regions) for the micelles. This leads to an entropy penalty that can be relieved if the CNTs aggregate, giving rise to the depletion attraction phenomenon. It seems to be of particular importance when using the commonly employed anionic surfactant sodium dodecyl sulfate (SDS) for aiding the dispersion, whereas the cationic surfactant hexadecyl trimethylammonium bromide (CTAB) turns out to be much less susceptible to induce depletion attraction [75, 76]. In fact, the beautiful cryo-TEM work of Nativ-Roth and co-workers revealed that SWCNTs dispersed in CTAB tend to align uniformly within small clusters at intermediate surfactant concentrations, well above the critical micelle concentration but below the threshold for development of a macroscopically ordered liquid crystal phase. In their most recent work [77], they also showed that this type of local nanotube alignment occurs also in suspensions stabilized by anionic surfactants of intermediate concentrations, or in suspensions combining ionic and nonionic surfactants. The mechanism for this unexpected local ordering is not yet entirely clear but the authors propose that van der Waals interactions between nanotubes coated by surfactants that form a hemimicellar structure around the tubes, giving a periodically varying charge density, may be at the origin of the phenomenon.
Nevertheless, even when SDS is used CNTs can be kept well dispersed over fairly long time in the lyotropic host [69, 71], most likely due to the high viscosity of the host phase. Furthermore, improvement was achieved by combining anionic surfactants for dispersing the nanotubes with cationic surfactants for forming the liquid crystal phase: since the excess micelles of the host phase are then attracted rather than repelled from the nanotubes, they will act more as 'buffers' against aggregation than as depletants. Using sodium dodecyl benzene sulfonate (SDBS) for dispersing the tubes and CTAB for forming the liquid crystal phase very good results were obtained [61, 66, 67], as will be further discussed below. Furthermore, interesting alternatives for dispersing CNTs in water for example, DNA [59, 66, 78–86] and bile salts [87, 88].
For thermotropic hosts other solutions are required. The problem is here that both nanotubes and host are non-polar, hence it is difficult to design an appropriate amphiphilic molecule structure. On the other hand, many thermotropic liquid crystals are actually surprisingly good solvents for carbon nanotubes [89, 90]. For achieving a first rough dispersion of the CNTs in a thermotropic nematic it is in fact sufficient to just stir the sample over the time scale of an hour (for high-quality results sonication is, however, required). Scalia and co-workers also found using Raman spectroscopy that the commonly used cyanobiphenyl mesogens interact with carbon nanotubes, as evidenced by a shift in the Radial Breating Mode (RBM) wavenumber [91]. In the first systematic study of CNT dispersion in thermotropic liquid crystals, Schymura et al. found that the performance of the commonly used nematic mixture E7 is fully comparable with NMP and the negative anisotropy mesogen n-(4-methoxybenzylidene)-4-butylaniline (MBBA) was even somewhat better [90], as shown in Figure 10.4. However, the exact molecular structure of the mesogen is very important, dispersion for example, in pure 5CB (the majority component of E7) yielding much poorer results. This study also revealed that the nematic state is beneficial to dispersion, hence one should not heat a carbon nanotube-doped thermotropic liquid crystal to its isotropic state. The time to nanotube aggregation is then drastically reduced.
Figure 10.4 Thermotropic liquid crystals show surprisingly good performance in dispersing SWCNTs at the early stage of sonication, as shown in panel (a). The light transmitted through the sample is monitored at the beginning of the tip sonication process. Decrease of transmission indicates that more and more CNTs are getting into the host, thus, absorbing more light. Isotropic solvents including an aqueous SDBS solution are used as reference. In (b) the LC molecular structures employed in the study are shown. Suspensions of SWCNTs in different LCs and in the isotropic organic solvent NMP used as reference are shown in (c) for different centrifugation times. E7 and MBBA behave comparably well to NMP. For further details. Reprinted with permission from [90].
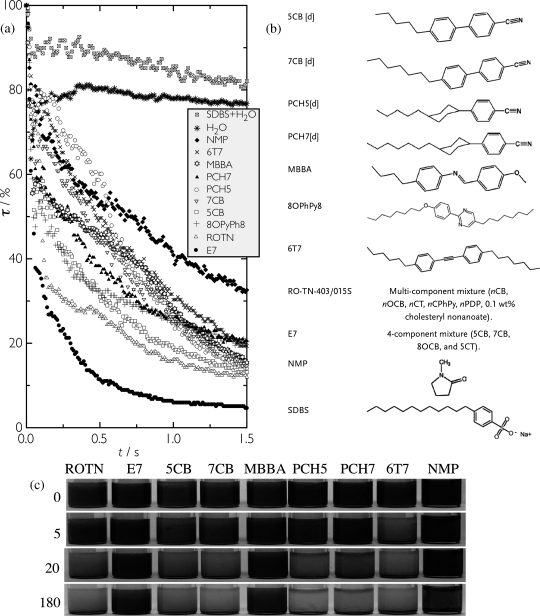
Despite the relatively good performance of some liquid crystals as CNT hosts, long-term stability of dispersions of pristine carbon nanotubes in a thermotropic liquid crystal has so far not been achieved. There is, thus, a clear need for new mediator substances that aid dispersion in non-aqueous solvents, specifically thermotropic liquid crystals. This approach is typically to combine a CNT ‘anchor unit’ with a moiety that interacts well with the host liquid, possibly through a spacer unit. The main difficulty lies in identifying an anchor unit that is truly effective in non-aqueous hosts, the most common choice being pyrene. Its similarity with a graphene sheet gives it the ability to adsorb strongly to the nanotube surface through aromatic interactions, minimizing the alteration of the electronic properties of CNTs. Meuer et al. used this moiety as anchor unit in their polymers tailor-designed for solubilizing MWCNTs in tetrahydrofuran (THF) [92]. The pyrene unit was attached with either a poly(methyl methacrylate) (PMMA) or a poly(ethylene glycol) (PEG) chain, rendering the nanotubes effectively ‘hairy’. At high-nanotube concentration the PEG–pyrene-solubilized nanotubes even formed a liquid crystal phase. Pyrene was also used as CNT anchor in other polymer mediator molecules specifically designed for aiding CNT incorporation into polymeric hosts [93]. We will discuss this work below in the context of CNT-loaded liquid crystalline polymers.
A very recent and highly interesting approach for improving the dispersion of nanotubes in liquid crystals is to synthesize molecules specifically designed for mediating between the nanotube and the liquid crystal matrix. This route has so far been explored by Kühnast et al. [94], synthesizing low-molar mass mediator molecules where a pyrene CNT anchor unit was linked through an oligoethylene spacer with a cyanobiphenyl mesogen, and by Kimura et al., who used an oligo(phenylenevinylene) mediator molecule terminated at both ends with cyanobiphenyl moieties [95]. In [94] dramatic improvement of the stability of SWCNTs dispersed in a standard commercial nematic thermotropic mixture was observed. The oligoethylene spacer unit turned out to be critical for the performance, mediator molecules with standard alkyl chains between pyrene and cyanobiphenyl units having almost no positive effect whatsoever. Most likely this difference is due to the fact that alkyl chains tend to adsorb strongly on a graphite-like surface [96]. The oligoethylene chain cannot do this; hence it is efficient in separating the cyanobiphenyl unit from the nanotube as it should. The efficient mediators exhibit no liquid crystalline phase on their own, in contrast to the variations with alkyl spacers which exhibited nematic and/or smectic mesophases. Whether this difference plays a role for the difference in effectiveness is not yet clarified.
The longer mediator molecule synthesized by Kimura et al. [95] does exhibit liquid crystal phases, of smectic type. It does not contain an anchor group, such as pyrene, but instead the whole conjugated oligomer wraps in a helical fashion around the nanotube efficiently, with the cyanobiphenyl termini promoting spontaneous liquid crystalline organization of the system. With the help of these new mediator molecules added at minimum concentration (the suspension was filtered after sonication to remove excess oligomers) the team could achieve very good nanotube suspension in chloroform, stable over several months. Interestingly, when they added more of the oligomer to the suspension, phase separation occurred, the nanotubes slowly precipitating into a phase which turns out to have the same liquid crystalline phase sequence as the pure oligomer. In other words, the nanotubes are incorporated into the liquid crystal phase of the oligomer used for stabilizing the suspension. Although no large-scale aligned samples were reported, the work is certainly very interesting and holds promise for future extensions. Unfortunately no mention is given as to whether the oligomer can stabilize a CNT suspension in a regular low-molar mass thermotropic liquid crystal.
A polymer mediator was used also by Lee et al. for dispersing nanotubes in organic solvents [97]. In their case the conjugated polymer P3HT, commonly studied in the field of organic electronics, was used for stabilizing nanotube suspensions in dichlorobenzene. This approach resulted in very good dispersions, with a maximum SWCNT loading of 2.75 mg/mL. In fact, these suspensions were so good that they spontaneously formed a lyotropic nematic liquid crystal phase, an issue we will return to below.
An alternative and more drastic approach is to functionalize the CNTs covalently, oxidizing them and possibly attach tailored moieties for enhancing compatibility with the matrix [98]. This approach has been particularly important in the field of CNT–polymer composites and we will thus discuss this approach briefly when discussing liquid crystalline polymers as hosts for carbon nanotubes. A related approach is the use of superacids for solubilising CNTs and forming lyotropic phases as will be discussed later.
Related to the issue of nanotube dispersion is the procedure to purify the nanotubes. No synthesis-based method produces only carbon nanotubes and the vast majority in fact leads to a quite substantial fraction of impurities. Thus, it is imperative for many applications that the nanotubes are purified. Often this is done by chemical as well as mechanical treatment, the latter typically being dispersion followed by centrifugation. Better-dispersed nanotubes remain in suspension whereas large-scale aggregates and impurities are largely collected in the sediment. When doing work on CNT–liquid crystal composites it can be quite important to consider the possible effects of impurities because many experiments (e.g. conductivity measurements) do not necessarily distinguish CNTs from certain impurities.
10.3 Liquid Crystal Phases of Carbon Nanotubes
An interesting processing route for obtaining ordered CNT materials is by induction of LC phases. This approach is technologically relevant because it is based on solution processing, quite attractive from an applicative point of view. The liquid crystalline phase is very important for the realization of extra-strong fibers of poly-(p-phenylenebenzobisoxazole), better known with its trademark name Kevlar. Therefore, LC phases can be useful for the realization of CNT materials with improved mechanical properties. Finally, CNTs can be used as model systems for studying the formation of LC phases of other anisotropic nanoparticles.
Carbon nanotubes, due to their elongated shape, are expected to form lyotropic liquid crystal phases when dispersed in liquids. The reason is based on the classical Onsager's theory of rigid rods forming liquid crystal phases at high-rod concentration [99, 100]. They indeed form LC phases as first shown by Windle and co-workers [101]. If the aspect ratio of the rods, that is, the ratio of the length and the diameter of the rods, and their concentration are high enough the free energy of the system is minimized if the rods form a liquid crystal phase. The loss in rotational freedom, that is, reduced orientational entropy, is compensated by a higher gain in translational entropy (the latter corresponds to a decrease of excluded volume). The formation of nematic lyotropic phases occurs above a threshold concentration, predicted by the Onsager theory being dependent on the dimension of the rods, specifically the volume fraction v = 3.3 d/L, where d is the diameter of the rods and L is their length. Research on LC phases formed by CNTs have been developed mainly by the Windle [101–103], Poulin [74, 80, 104], and Smalley– Pasquali [105–107] and co-workers using multi-wall CNTs as well as single-wall CNTs. Based on Onsager's theory we can estimate the amount of SWCNTs needed considering a typical SWCNT density of 1.5 g/mL and a SWCNT d/L ratio of 10–3. We would thus expect nematic phase formation already at about 0.5 wt.% loading. Since the aspect ratio of MWCNTs is lower than SWCNTs, we would expect for MWCNTs some ten times higher loading required. The reported experimental results show that only MWCNTs [101–103] come close to the theoretical predictions by Onsager, while higher loading of SWCNTs is needed for the formation of LC phases [80, 104] with some exceptions [104, 108] coming close to the theoretical prediction of 0.5 wt.%. There are several reasons that can influence the discrepancy between theory and experiments. One important problem is the difficulty in completely separating the nanotubes from each other: they tend to form ordered bundles that, as an overall, clearly possess a lower aspect ratio than isolated tubes. Thus, even if the average SWCNT d/L ratio may be on the order of 10–3, the aspect ratio of bundles can be substantially lower affecting then the loading of nanotubes needed for the formation of the LC phase. When successful dispersion of individual tubes has been achieved, this has in almost all cases been at the cost of severe cutting of the tubes, a typical negative side effect of the high-power sonication or of aggressive chemical treatments required for breaking up the CNT aggregates or preventing their re-aggregation.
In the Onsager theory the rods are not interacting and the only interaction is of the steric type. This is absolutely untrue for carbon nanotubes that strongly attract each others because of van der Waals forces. If the nanotubes are chemically treated or if there are surfactants or other type of molecules wrapping them up to counteract aggregation, the analysis of the forces entering into play becomes even more complex, not being usually possible to consider a balancing off with the attractive forces. The surfactants not only introduce repulsive electrostatic forces but also form a surrounding layer that together with the consequent double layer alter the effective dimension of the nanotube. Considering the diameter of the nanotubes, which is in the nanometer range, the “effective” diameter can be several times the original CNT diameter. On the one hand, the surfactant encapsulation increases the effective volume fraction considerably (at constant SWCNT weight fraction), on the other it does so at the expense of a great reduction in aspect ratio (a single surfactant molecule is typically twice as long as the SWCNT is thick).
The main result anyhow is that in general the interaction forces are all but negligible, playing a fundamental role in keeping the nanotubes apart or attracting them. Attractive forces exist in bare nanotubes promoting aggregation but repulsive forces are used in CNT dispersion for avoiding their reaggregation to achieve a high quality dispersion for example, a high loading with individual tube dispersion. Interestingly each of the three main groups active in the field has initially chosen its own approach for the dispersion of CNTs. Whereas Windle and co-workers oxidized their MWCNTs to make them water soluble [101–103] the Smalley and co-workers dispersed pristine SWCNTs in super acids [105–107, 109]. They have also used chlorosulfonic acid to dissolve SWCNTs, DWCNTs, and MWCNTs from different sources obtaining individual tube dispersions. They have observed that tubes with defects disperse worse and only the higher quality tubes were entering in the formation of LC phases [110]. Moreover, LC phases were also observed for longer SWCNTs as well MWCNTs (100 and 500 μm long, respectively) unlike other existing studies. The Poulin approach was instead to use DNA [80] or hyaluronic acid [104] for dispersing SWCNTs. In all cases nematic LC phases were achieved as verified by birefringent textures of the fluid suspensions detected in polarizing microscopy. Windle et al. also imaged disclinations in the nematic director field beautifully using scanning electron microscopy on samples from which the solvent had been evaporated [101, 103, 111]. The liquid crystalline structure remained intact upon drying. In this way they could have a closer look at the local arrangement and follow the director orientation visualizing almost the constituent building blocks of the LC phase. The authors found ½ disclinations with both sign, namely +1/2 and –1/2 disclinations.
The Onsager theory was developed for monodisperse rods, meaning rods possessing the same dimension. This assumption is not valid for typical samples of CNTs. A treatment taking polydispersity into account reveals that at a certain rod concentration a biphase region isotropic + nematic appear with the rods of proper dimension and straightness forming the LC phase while the curved or too short rods will segregate into the isotropic region [100, 103], cf. Figure 10.5. This behaviour could be interesting to obtain size fractionation of the nanotubes according to the length [103]. After centrifugation the isotropic phase will be at the upper part while the nematic is on the bottom. The supernatant can then be removed and the procedure can be repeated several times leading to a narrower and narrower nanotube length distribution. This procedure is feasible on lab scale and is scientifically very interesting, but it might be too complex and time consuming to be applied industrially.
Figure 10.5 Size segregation of CNTs induced by phase separation. In the left image isotropic phase composed of shorter and probably more defected CNTs. On the right CNTs with dimension and quality suitable for the formation of LC phases. Source: Reprinted with permission from Zhang et al. [103]. Copyright (2006) American Chemical Society.
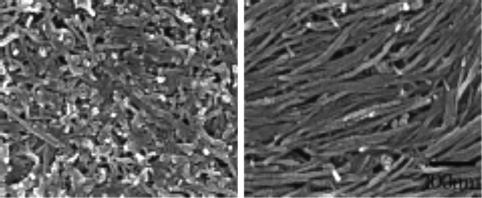
The threshold value for the formation of the nematic LC phase was estimated by Song et al. [101] from the concentration of nanotubes needed for reaching percolation of droplets of nematic phase, within an isotropic background, to be around 4.3 vol.%. The transition from the isotropic to the liquid crystal phase was also studied for SWCNT suspensions [107]. The corresponding textures were again recognized by polarizing optical microscopy. A wide range of isotropic–nematic phase coexistence was observed by both groups. As discussed previously the reason for the bi-phase region lies in the polydispersity of size of carbon nanotubes [51, 112]. The higher the CNT polydispersity, the wider the region of phase coexistence.
Another important divergence from the theory is related with the rigidity: Onsager theory assumes perfect rod rigidity which is not at all true especially for long nanotubes. This would suggest that the formation of LC phases would not be realizable for very long and thin nanotubes. The relation between size of carbon nanotubes and formation of LC phases is investigated by Song and Windle [111]. Systematic studies with nanotubes of different length and diameters would be highly desirable but not currently feasible due to the lack of availability of such highly size-controlled samples. The authors investigated three samples of multi-walled CNTs not finding a straightforward relation between aspect ratio and threshold value of concentration for formation of LC phases. However, the texture and the density of defects of the resulting nematic phase were very different indicating a strong effect of the dimensions of the CNTs on the LC phase. They could distinctly observe that shorter and thicker CNTs, having also the lowest aspect ratio, aligned in a more continuous and packed way than the others, resembling ordinary nematics. Interestingly, for the other two cases, that is, MWCNTs with higher aspect ratio and with greater length, respectively, the authors observed a bending of the nanotubes themselves around the defects instead of the typical change of orientation of the director resulting from the almost continuous change of direction of straight rods. The density of defects was also different in different samples. This outlines the importance of not only the aspect ratio but also of each dimension of the rods.
In terms of applicability, a drawback with the method of inducing LC phase behavior directly in CNT suspensions is the control of the director orientation, a prerequisite if one wants to use the method to deposit CNTs on a substrate along specific directions. The drying process can influence the alignment of CNTs as shown in Ref. [113] but even if scientifically interesting this does not appear straightforwardly usable for large scale alignment. Shear flow can induce alignment and this can be frozen in fibers with a high degree of alignment, as evidenced by the successful solution spinning of carbon nanotube fibers from a liquid crystal phase of CNTs [11, 51, 106, 109, 114, 115]. Zamora-Ledezma et al. [68] have shown that it is possible to impose a common unidirectional orientation in films also by shearing SWCNT lyotropic LCs, preserving the order parameter in the sample after drying. Their thorough analysis of the anisotropic properties demonstrates not only the achieved uniform alignment but also that their typical order parameters are in the order of 0.1–0.15. This value should be compared to the expected value from Onsager's theory at isotropic–nematic phase transition being <0.79. The much lower value observed experimentally outlines the high complexity of typical CNT systems and of SWCNTs in particular due to the possible formation of viscoelastic systems and to the tube “waviness” rather than rigidity.
The importance of the straightness and rigidity of SWCNTs for forming LC phases was further investigated by Puech et al. [108]. Initially their SWCNTs did not show a real tendency to form ordered phases because of the conformation of the starting SWCNTs comprising defected tubes with branches or kinks and long tube that would not keep themselves straight. After systematic sonication and centrifugation steps they obtained shorter tubes that formed LC phases with a dramatically improved order parameter. In dried samples they obtained a value of S = 0.55. In Figure 10.6a the tubes are shown after a first sonication and centrifugation treatment, exhibiting branches and bending while in Figure 10.6b after long treatment they appear shorter, thinner, and straighter. Phase transitions can be used for sorting CNTs segregating the shorter or less straight and perfect into the isotropic phase. This strategy was used for further enriching the LC phase with tubes with size in the fluid phase and shape more suitable for building up LC phases. In this way the authors could measure the order parameter in the nematic regions, surrounded by the isotropic phase, forming droplets in shape resembling the tactoids, discussed theoretically in Ref. [88]. In these islands the order parameter was distinctly higher, reaching the value of 0.65, closer to the value predicted by Onsager theory. This work confirms the importance of the quality and dimension of the CNTs for being good “building blocks” of LC phases.
Figure 10.6 TEM images of CNTs. In (a) they were sonicated for 30 min and ultracentrifuged for 45 min while in (b) the sonication was performed for 3 h followed by 3 h of ultrascentrifugation. The size of the image is 1.5 × 1.5 μm2. Source: Reprinted with permission from Puech et al. [108]. Copyright (2011) American Chemical Society.
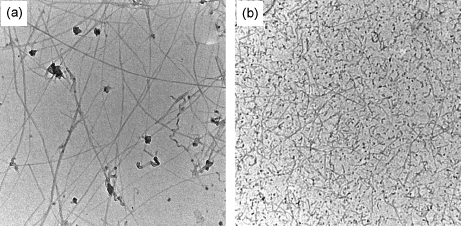
The above mentioned work by Lee et al., in which SWCNTs were suspended in dichlorobenzene with the help of the conjugated polymer P3HT [97] is interesting because it constitutes also a case of nematic lyotropic liquid crystal formation by the nanotubes where a uniform alignment could easily be achieved over a large monodomain by shearing. The authors could confirm the uniform alignment of the nanotubes by atomic force microscopy and polarized optical absorption spectroscopy. Moreover, they placed the thermotropic nematic liquid crystal 5CB in contact with a film prepared from the shear-aligned lyotropic CNT suspension, achieving uniform alignment of the 5CB director along the nanotubes, that is the nanotube film acts as alignment layer for the thermotropic nematic director.
A slightly different approach to achieving lyotropic liquid crystal formation from CNTs was presented by Islam et al. [116]. They prepared low-concentration nanotubes suspensions in an N-isopropyl acrylamide gel and then induced the ordering through a volume–compression transition. Although the resulting films are actually not fluid any more (they are in a gel state) they can be discussed in terms of nematic ordering. The ordering of the nanotubes can be quite impressive, reaching orientational order parameter values of about 0.8.
10.4 Carbon Nanotubes Aligned by Thermotropic Liquid Crystals
Inclusions of rods with ferromagnetic properties, in thermotropic LCs where investigated first theoretically envisaging a possible improvement of the reorientational LC properties [117, 118]. These rods had all dimensions large enough compared with the LC molecules to be successfully described within the continuum theory. This situation is quite different from the inclusion of CNTs that have length much larger than the LC molecules but diameters in the nanometer range. Interestingly, the research on CNTs in thermotropics has evolved in the opposite way compared to the early seventies, starting, and developing mainly with experimental works, with some already mentioned theoretical exceptions.
The effect on phase transitions, optical, photorefractive, and electro-optic properties of LCs have been widely investigated [46, 89, 98, 119–140]. The vast majority of works in the field deals with nematics with some exceptions [89, 29, 130, 141–145]. In a recent series of experiments conducted by Rosenblatt and co-workers [142–144] multiwall carbon nanotubes were explored in their role as chiral dopants of liquid crystals. The authors found small but nevertheless clear-cut evidence of chirality transfer from the nanotubes to the liquid crystal both in smectic and nematic host phases. If the chiral species (here the nanotubes) is present with perfectly equal amounts of left- and right-handed enantiomers, that is, as a perfect racemate, such effects cannot occur. Usually carbon nanotube samples are considered as racemic because the synthesis procedures should not introduce any asymmetry and since any type of CNT chirality control is in fact extremely challenging. The results of the Rosenblatt team indicate, however, that at least this source of multiwall carbon nanotubes produces the tubes with a slight enantiomeric excess. Since, the scope of this book is non-display applications, we will hereby focus on the use of thermotropic liquid crystals for CNT organization. In fact, using thermotropic LCs as host the liquid crystalline organization can be transferred to the nanotubes. The transfer of order is a very interesting and effective way to align CNTs permitting to explore routes towards aligned.
CNTs are notoriously difficult to organize thus their control through a matrix is an attractive and innovative strategy. If arrays of aligned tubes are desired thermotropics can be more awkward to use even if attempts towards a matrix free use was made by Lynch and Patrick [146]. Alternatively thermotropics can open the route toward polymerized systems or CNT manipulation in fluid environment. Besides, the alignment of CNTs in LCs with the transfer of order is an intriguing scientific topic on its own.
The dispersion in thermotropics has some peculiar aspects in terms of the mechanism acting between tubes that can be beneficial for the dispersion [90]. In general thermotropics can accept lower concentrations of CNTs than lyotropics before aggregation. This disadvantage is compensated by the net advantage of well-developed alignment methods due to the display industry and an ease of uniform alignment and of reorientation. Thermotropics are therefore very attractive tools for manipulating carbon nanotubes.
The experimental studies have indicated so far an orientation of CNTs in nematics mainly along the director. Because of the exceptionally high electrical conductivity of the CNTs it is reasonable to investigate this property in the LC–CNT composite to infer information on the orientation of CNTs. The change in conductance, while switching the LC in a cell, was monitored and analyzed in Refs [147, 148]. Electrical investigations are, however, only an indirect proof of the CNT alignment and the data rely on the change of conductivity between undoped and doped samples solely due to CNTs. However, in normal samples, there are other impurities that can contribute substantially to the electrical response.
Because of the nanoscopic dimension of CNTs the direct assessment of their orientation is not trivial. Lynch and Patrick have shown unidirectional alignment of CNTs in nematic LCs (5CB and E7) visualizing the alignment by atomic force microscope (AFM) after removal of the host [146], cf. Figure 10.7. They had deposited a composite of CNTs and nematic LC on a rubbed filter membrane. Through the porous membrane the excess of LC was removed leaving the CNTs on the substrate. The nanotubes could then be visualized by AFM showing a unidirectional alignment of the CNTs along the rubbing direction. Depositing electrodes they could induce an in-plane reorientation of the LC and also of the CNTs. From the orientational distribution of the MWCNTs they obtained a value for the orientational order parameter, evaluated for a 2D system (the CNTs are in the substrate plane) ranging between 0.7 and 0.9, a substantially higher value than for typical nematics. Their method has the advantage of removal of the matrix and the direct observation of the CNTs. However, it is desirable to investigate the alignment of CNTs also within the bulk monitoring the sole effect of LC ordering without any additional step or forces that could interfere with the process.
Figure 10.7 MWCNTs on a filter membrane aligned by the LC visualized by AFM after LC removal. Source: Reprinted with permission from Lynch and Patrick [146]. Copyright (2002) American Chemical Society.
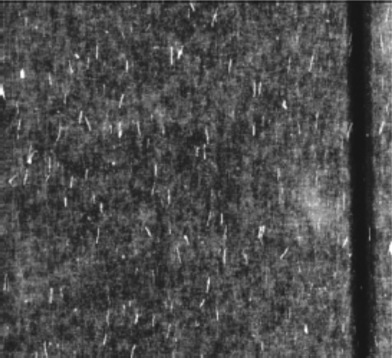
An unambiguous way to access the alignment of SWCNTs is through polarized Raman spectroscopy [149]. SWCNTs have a characteristic Raman spectrum that allows to recognize and distinguish the presence of SWCNTs. Because electronic transitions of SWCNTs are usually resonant with laser wavelengths, a decent Raman signal can be detected also from minute quantities of CNTs due to a resonance effect. The most intense mode in the SWCNT spectrum is the G-band, visible at a wavenumber of about 1590 cm−1, whose two main peaks are due to tangential vibrations parallel to the long axis and tangential to the circumferences respectively. If the tubes are defected or carbon impurities are present in the sample a band designed with the letter D, at around 1350 cm−1, becomes more pronounced. A mode specific of SWCNTs is the radial breathing mode (RBM) arising from the tubular structure and based on radial vibrations. It has been shown that by using polarized light it is possible, the first step in the Raman process, to discriminate the orientation of CNTs because the light absorption of CNTs is anisotropic, being much higher along the tube axis [149]. Thus, by monitoring the intensity of the Raman peaks it is possible to discriminate the orientation of the CNTs. Investigations in a standard LC cell filled with 5CB and SWCNTs of the HiPco type were performed analyzing the most intense peak, the G band, for polarization parallel and perpendicular (figure 10.8) to the LC director. The decrease of peak intensity from the first to the second case gives a clear, unambiguous proof of the alignment of SWCNTs along the LC director in the LC steady state [46, 150].
A difficulty might come from modes of some LC molecules, especially the ones with aromatic core, with peak positions very close to the G band. However, as shown in Figure 10.8, the G band can often be visualized despite the LC peaks when the peak positions are sufficiently separated. Raman spectroscopy is very informative concerning CNT quality, orientation, doping, bundling, interaction with the environment, etc. More information can be deduced by the Raman spectrum of SWCNTs but MWCNTs can be analyzed as well. The RBM is sensitive to the environment and can show a shift of its position also for noncovalent interactions like π–π stacking as shown in Figure 10.9b.
Figure 10.8 Alignment of SWCNTs probed by analyzing the change of intensity of the Raman modes for different directions of light polarization. Source: Reprint with permission from Scalia et al. [46].
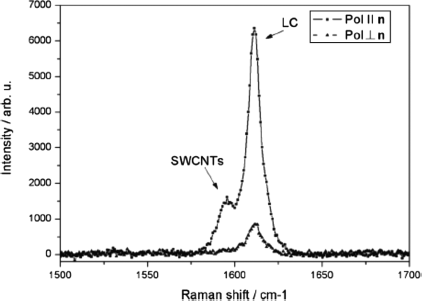
Figure 10.9 (a) Transition nematic–isotropic (left) and isotropic–nematic (right) around bundles of SWCNTs. (b) Shift of the position of RBMs between pristine and embedded in LC SWCNTs. Source: Reprinted permission from Scalia et al. [91].
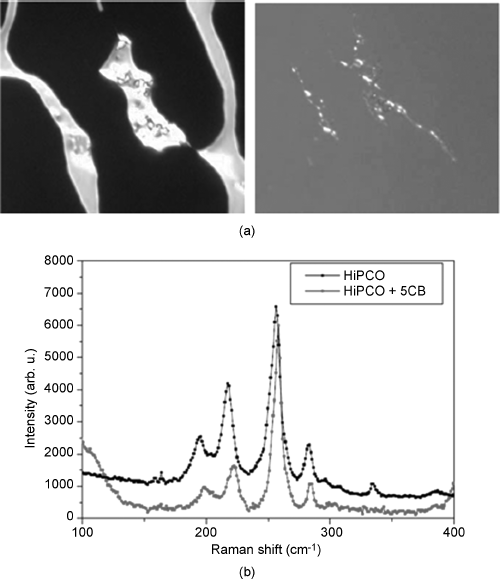
The interaction between LC molecules and CNTs is quite important because it could affect the reciprocal orientation and presumably the reciprocal degree of order. The direction of LC orientation and the strength of the anchoring onto surfaces is fundamental for the alignment of LCs in cells and it is expected to be relevant also for the combination with CNTs. The graphitic structure seems quite suitable for the development of strong π–π interactions with aromatic LCs. From the dispersion study of Schymura et al. [90], it is clear that the aromaticity is not the only key aspect for the establishment of an interaction at the surface but the molecular structure of the LC on the whole indeed plays a role. The ease to disperse CNTs at the first stage of the mixing is not the only way to visualize the interaction. Around large aggregates of SWCNTs in 5CB an increase of stability of the nematic phase was observed at the nematic–isotropic phase transition [91], cf. Figure 10.9a. Vice versa, coming from the isotropic phase the nuclei of nematic phase appear at the tube interface. The CNT surfaces thus appear to be a suitable interface for LC anchoring. In addition the effect of the interaction can be seen also from the shift of the Raman RBMs of the tubes between doped and undoped samples, cf. Figure 10.9b.
10.5 Carbon Nanotubes Aligned by Lyotropic Liquid Crystals
Kumar et al. [151], first reported the use of lyotropic liquid crystals as aligning hosts for carbon nanotubes, where poly(p-phenylene benzobisoxazole) (PBO) was synthesized by in situ polymerization in the presence of single-wall CNTs in poly(phosphoric acid) (PPA). The resulting solutions, with CNT to polymer concentrations of 0%, 5%, and 10%, form a nematic lyotropic liquid crystalline phase which the authors could spin into fibers. Optical microscopy images suggest good dispersion of the tubes (although the sole image shown is obtained with the sample between crossed polarizers, making it difficult to recognize nanotube aggregates) and the mechanical properties of the fibers were substantially enhanced by the presence of the CNTs. The tensile modulus, tensile strength, and elongation to break were found to be 20%, 60%, and 40% better, respectively, than for CNT-free PBO fibers. For instance, tensile strengths of pure PBO fibers were in the range 1.8–2.6 GPa whereas the fibers with 10% nanotubes showed tensile strengths in the range 2.9–4.2 GPa. Considering the rather large amount of nanotubes the improvement must still be regarded as a disappointment, probably due to insufficient dispersion quality (the nanotubes were pristine SWCNTs and no mediator was added) and/or to insufficient adhesion between the matrix polymer and the nanotube surfaces. No increase in electrical conductivity was measured for the fibers, a result that the authors attributed to the good alignment of the nanotubes, leading to a high-percolation threshold. However, the lack of electrical percolation, which is in fact quite surprising at such high-nanotube concentration, may equally well be due to insufficient dispersion quality, preventing a continuous conduction path through the polymer because the nanotubes are aggregated instead of well distributed throughout the sample. Indeed, later works on liquid crystalline polymers loaded with nanotubes (see below) report similar enhancements of mechanical properties at an order of magnitude lower nanotube concentration, suggesting that this early work did not represent optimum conditions.
Li et al. revisited the CNT–PBO system in 2006 [152], this time using oxidized MWCNTs and polymerizing in situ with the CNTs added to the PPA solvent together with the monomers. The oxidation of the CNTs allowed for better dispersion as well as covalent incorporation in the polymer during polymerization. Also in this work the authors spun fibers from the lyotropic nematic solutions, getting somewhat better results. Compared to the pure PBO fibers, composites with 2 wt.% MWCNTs had tensile strength improvements in the range 20–50%. The orientational order of the CNT-loaded fibers was, however, less than that of pristine PBO fibers, suggesting that the dispersion of the nanotubes was still not satisfactory.
A more recent paper that also used a polymer-based lyotropic liquid crystal host is that of Okano et al. [153]. In contrast to the work of Kumar et al. the system of Okano and co-workers has the great advantage of being water-based, the polymer used for dispersing the nanotubes as well as for forming the lyotropic phase being sulfonated polyaramide (PPSA). This polymer forms a nematic phase already at a concentration of 0.6 wt.% and its anionic amphiphilic character gives it a good CNT dispersion capability: for a single-wall nanotube concentration of 1 mg/mL (0.1 wt.%) no CNT aggregates could be detected by optical microscopy in the lyotropic composite and the dispersions were reported to be stable for at least a year. The CNT-containing lyotropic system was spread on a substrate using a bar coater, inducing a uniform alignment of the liquid crystal director over a large area. An important aspect is that the water could be evaporated, leaving behind a solid system, without loss of the orientational order. The authors used polarized Raman spectroscopy to evaluate the orientational order of the nanotubes, finding a value for the order parameter of the SWCNTs of 0.48.
Most work on lyotropic liquid crystals as hosts for carbon nanotube dispersion and alignment has, however, been done using low-molar mass surfactants forming the host phase. This approach is attractive because one can use the surfactants employed as standard procedure for dispersing carbon nanotubes to form the lyotropic liquid crystal host phase. While composites of carbon nanotubes in thermotropic nematic are often done by introducing the nanotubes directly into the liquid crystal, this approach is rarely followed for the case of lyotropic host phases, which are typically inappropriate as host for carrying out the initial dispersion. Presumibly they are too viscous to achieve efficient sonication. Neither the surfactant nor the water has any dissolving power on the CNTs [90]. The surfactant wrapping and the water solvent simply keep the nanotubes dispersed, but the actual separation of the tubes must be done by mechanical methods, normally sonication.
The starting point for preparing a surfactant-based lyotropic liquid crystal–CNT composite is, thus, generally a high-quality isotropic CNT suspension in water, stabilized by a standard surfactant, such as SDS or CTAB. The transition to a lyotropic liquid crystal can then follow a number of different routes. In the earliest reports the isotropic CNT suspension was either mixed with a liquid crystalline surfactant solution of very high concentration, such that the desired surfactant concentration resulted in the final mixture [70], or dry surfactant and co-surfactant were added to the nanotube suspension to increase the surfactant concentration to the threshold for a nematic phase [69, 71]. In these first experiments on lyotropic liquid crystal-driven nanotube alignment the CNT concentration was rather low, rendering confirmation of the successful transfer of orientational order non-trivial. The first clear-cut evidence of alignment was provided by using polarized Raman spectroscopy [69, 71], giving unambiguous proof that SWCNTs were indeed oriented along the director of the lyotropic phase. The concept worked even upon addition of a chiral dopant rendering the host cholesteric (chiral nematic) with a pitch of its helical director modulation in the 100 μm range.
An important improvement was achieved by combining cationic and anionic surfactants, one for stabilizing the nanotube suspension, the other for forming the liquid crystal phase [67]. The cationic surfactant CTAB was added at sufficient concentration to form the desired liquid crystal host phase to a prepared high-quality suspension of CNTs stabilized by anionic SDBS. In this way very high concentrations of nanotubes could be introduced to the liquid crystal phase with retained orientational order, such that the macroscopic phase behaved as a fluid polarizer, cf. Figure 10.10: the orientationally ordered nanotubes absorb light polarized along the tube axis, but not light with the perpendicular polarization. Thus, while the uniform alignment was corroborated with polarized Raman spectroscopy also in this work, the fact that the director of the liquid crystal phase could easily be uniformly aligned over square centimeter areas by shearing allowed the transfer of uniaxial orientational order to the nanotubes to be verified by simple inspection by eye through a linear polarizer. Importantly, the method combining cationic and anionic surfactants works equally well for single-wall CNTs [67] as well as for multi-wall CNTs [66], whereas other work with lyotropic liquid crystal hosts reported ordering only for single-wall but not for multi-wall nanotubes, presumably because the MWCNT diameter is much greater than that of the native lyotropic phase micelles [65, 75]. In the cationic system each nanotube is most likely surrounded by a ‘buffer layer’ of oppositely charged native micelles. This will reduce the risk of depletion attraction and probably improve the incorporation possibilities in the host phase, even when the nanotubes are of a different diameter than the host micelles.
Figure 10.10 Two capillaries filled with single-wall carbon nanotube suspensions at the same concentrations, in one case in an isotropic host (left capillary) and in the other in a uniformly aligned liquid crystal host (right capillary, director along capillary axis). The samples are viewed with illumination from below through a linear polarizer held with its absorption direction (double arrow) perpendicular (left image) or parallel (right image) to the capillaries. For the isotropic sample this induces no change but the liquid crystal sample is much darker with the absorption direction parallel to the capillary than perpendicular. This is because the CNTs absorb preferentially light polarized along the tubes, that is, along the capillary in the liquid crystalline sample, leaving less light to go through the polarizer in the right image than in the left image. In the isotropic samples the nanotubes are randomly oriented, thus absorbing light equally strong for any polarization. A standard metric ruler is placed along the top edge of the photos to show the scale.
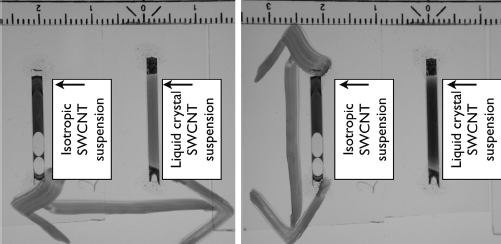
The catanionic CNT suspension also exhibited very interesting rheological properties, with thin filaments in which the nanotubes are highly aligned being easy to draw from the phase [61, 67]. This phenomenon that occurs neither for the CNT-free liquid crystal phase nor for isotropic nanotube suspensions, can be understood as a percolation-like process where the micelle length diverges during elongational flow as a result of a positive feedback loop between orientational order, micelle stiffness, and length [61]. It happens for single-wall as well as multi-wall carbon nanotubes of different types but not for fullerenes and also not if the dispersion quality of nanotubes is insufficient. Recently, the rheological properties of nanotubes suspended in purely anionic SDS-based nematic lyotropic phases were investigated thoroughly by Tardani and Camillo [62]. They found significant viscoelastic behavior at nanotube loadings above 0.25%. The behavior was explained as a result of nanotube entanglement within the nematic matrix.
Xin et al. recently introduce an attractive alternative approach to forming the CNT-loaded lyotropic liquid crystal phase [63, 65]. As customary they started with an isotropic low-surfactant concentration suspension of nanotubes, stabilized either by nonionic dodecyl octaoxyethene monoether surfactant [65] or by an ionic surfactant (either CTAB or SDS) [63], but the liquid crystal phase formation was triggered by adding a large amount of polymer, inducing depletion attraction. For nonionically dispersed nanotubes nonioinic poly(ethylene glycol) (PEG) was added, while for CNTs stabilized by ionic surfactants a polyelectrolyte of the same charge as the surfactant was introduced. Due to the depletion attraction the surfactant-wrapped nanotubes aggregate, together with polymer and surfactant, forming a hexagonal columnar liquid crystal phase in which they are apparently still separated from each other. This phase separates from an essentially nanotube-free phase containing only polymer in water solution. The beautiful aspect of this approach is that the nanotube concentration in the liquid crystal phase is substantially raised compared to the initial suspension. An important question that remains to be addressed, however, is if the hexagonal phase can be aligned macroscopically. The pictures shown in these papers were from a polydomain sample, confirming the lyotropic liquid crystalline nature but not that the nanotubes were uniformly aligned. Moreover, the lack of high-magnification microscopy images taken without crossed polarizers precludes a true confirmation that no bundling of the nanotubes occurred.
Most work with lyotropic hosts has been done with aqueous solvents, although some exceptions exist, such as the already mentioned PPA-dissolved PBO. Another example is the report by Jiang et al. [154] where an ionic liquid was used as solvent for nanotubes dispersed with a nonionic polyoxyethylene surfactant. As in the works of Xin and co-workers the liquid crystal phase was of hexagonal columnar type. The same difficulty in obtaining a macroscopically uniform orientation of the host phase was probably the reason why no attempt to verify the alignment of the CNTs was reported in this work. The only case known to the author where a uniformly aligned purely hexagonal liquid crystal phase loaded with carbon nanotubes was prepared and investigated is the recent work of Mauter et al. [155] (The cationic system described by Scalia et al. [67] also formed a hexagonal columnar phase, as confirmed by X-ray scattering, but subsequent investigation showed that this phase occurs very close to a nematic phase, which probably prevails during shearing, making the uniform alignment by shearing of this phase possible.) As in the work of Scalia et al. [67] a combination of cationic and anionic surfactants was used, here sodium taurodeoxycholate for suspending the CNTs and CTAB for forming the hexagonal columnar liquid crystal phase. The authors took great care in aligning the phase uniformly by cooling the mixture slowly from the isotropic temperature range to that of hexagonal columnar order in the presence of a 5T magnetic field. A very interesting aspect of this work is that the team polymerized the system after alignment, resulting in a solid film with integrated aligned nanotubes. Polarized Raman spectroscopy was used to verify that the nanotube orientation had not been lost in the process, and indeed the contrast between the responses for polarization parallel and perpendicular to the director was excellent. The nanotube concentration was rather low in this work (well below 0.1 wt.%), but if it can be increased in future work this approach holds great promise for manufacturing films profiting from well dispersed and uniformly aligned carbon nanotubes.
10.6 Carbon Nanotubes in Liquid Crystalline Polymers or Polymerized Liquid Crystals
The alignment of the nanotubes imposed by liquid crystallinity is very useful in the context of polymeric matrices. In contrast to low-viscous and low-molar mass liquid crystal hosts that must be contained in vials or cells, a liquid crystalline polymer can be self-sustaining, often forming a glass with retained orientational order at room temperature. By spinning a fiber from the composite, impressive mechanical properties can be achieved. Compared to CNT composites with low-molar mass liquid crystals, a very important additional aspect in case of composites with liquid crystal polymers is—as in the case of any polymer–CNT composite—the adhesion between CNTs and polymer matrix, important for an efficient load transfer. Very often this is inadequate, resulting in nanotubes sliding within the matrix upon mechanical stress, thereby cancelling any beneficial mechanical effect from the CNT addition, regardless of the quality of their alignment and dispersion. Related to the use of liquid crystalline polymers is the approach to disperse the nanotubes in a reactive low-molar mass liquid crystal host and then polymerize the composite, resulting in a solid material with incorporated aligned carbon nanotubes.
As discussed above, dispersion is an immense challenge in preparing nanotube—liquid crystal composites, and the problems are if possible even greater when the host is polymeric. Simply mixing pristine nanotubes in a standard liquid crystalline polymer, molten or dissolved, will typically yield poor results, with substantial nanotube aggregation. Nevertheless, this approach was attempted by Choi et al., mixing up to 2 wt.% of pristine multiwall CNTs in the aromatic copolyester Vectra A950 using melt compounding [156]. The CNTs had no significant effect on the transition temperatures of the Vectra host, an observation that may be seen as a sign of weak interaction between the matrix and the filler particles. Despite the lack of CNT treatment to improve the compatibility with the host an improvement of mechanical properties was reported. With 2% of MWCNTs added the tensile strength increased from 62.3 MPa to 89.3 MPa (43.3% increase) and the modulus increased from 1.12 GPa to 1.79 GPa (59.8%). In principle the authors had expected even greater improvement and they attribute the limitations to insufficient dispersion of the nanotubes in the matrix. The authors did not assess the alignment of the tubes in the Vectra matrix. Another team attempted to polymerize the monomers in situ in the presence of pristine MWCNTs [157], yielding a nematic polymer–CNT composite. The characterization of this composite was not very detailed so it is difficult to judge if the in situ polymerization improved the situation compared to the approach to mix the CNTs directly with the pre-existing nematic polymer. Spectroscopy and microscopy studies suggest that the interactions between liquid crystal polymer and nanotubes were reasonably strong but further studies are required to assess the success of this approach.
There are essentially three different approaches that have been explored to circumvent the problems with dispersion of the CNTs in liquid crystalline polymers: (i) functionalize the nanotubes covalently for improved compatibility with the polymer; (ii) add a tailormade molecule that adsorbs noncovalently on the nanotube and mediates between filler and matrix; or (iii) modify the liquid crystal polymer for enhanced compatibility with the nanotubes. Although it generally impairs the properties of the nanotubes, at least for SWCNTs, the most common choice for realizing attractive CNT–liquid crystal polymer composites is the first one, to covalently functionalize the nanotubes. Either they are simply oxidized resulting in carboxylic acid groups attached to the CNT sidewalls [158, 159] or one grafts more complex moieties to the nanotubes, specifically designed to enhance compatibility with the host [158–160]. Sahoo et al. compared carboxylate group and hydroxyl benzoic acid (HBA)-functionalized MWCNTs for incorporation in a liquid crystalline polymer (the authors did not specify which phase the polymer formed) consisting of 80% HBA and 20% ethylene terphtalate (ET) [159]. The HBA-functionalized nanotubes are expected to be particularly easy to disperse because the covalently attached groups are identical to the majority component of the liquid crystal polymer. The nanotubes were introduced into the polymer at a concentration of 1 wt.% by mixing in a microcompounder, heated to a temperature above the melting point of the polymer. Absorbance spectroscopy revealed a non-negligible shift of the peaks for the two functionalized CNT types, in contrast to when raw MWCNTs were added to the polymer, indicating an enhanced interaction between the matrix and the nanotubes after these had been functionalized according to either route. The improved interaction was corroborated by scanning electron microscopy investigation of fractured surfaces, revealing nanotubes that had been broken upon fracture rather than pulled out of the matrix. Optical microscopy investigations also showed that the functionalized nanotubes were substantially better dispersed than the raw nanotubes, although all composites exhibited aggregates that were easily visible on the optical scale, that is, they were on the size of several microns. Mechanical testing of the composites also revealed a beneficial effect of the nanotube functionalization, the greatest improvement being seen for HBA–CNTs, as expected. Here the tensile strength increased from 87.5 MPa for the neat liquid crystal polymer to 123.5 MPa (41% increase) and the tensile modulus increased by 55%, from 2.9 GPa to 4.5 GPa. While the authors found substantially lower degree of improvement for the pristine CNTs (6% and 28%) it is somewhat surprising that their reference values are much poorer than the above mentioned data for pristine nanotubes from Choi et al. [156]. Although the concentration of nanotubes differed by a factor of 2 between these studies the differences indicate that variations in the chemical structure of the polymer may be just as important as nanotube functionalization.
In the study of Lafuente et al. [158] three different functionalizations of single-wall CNTs were compared for integration into a nematic polyazomethine host. In addition to the standard carboxylate group attachment the authors prepared SWCNTs functionalized with CONH(CH2)6NH2 amines or with 2-methyl-1,4-phenylenediamines. These two functionalizations are optimized for the target liquid crystal polymer host because the amine terminus can link covalently to the monomers of the host polymer. In fact, the 2-methyl-1,4-phenylenediamine constitutes one of the two monomers used for forming the liquid crystalline polymer. The SWCNTs were mixed at 1 wt.% concentration with the monomers and then the polymer was prepared by in situ polycondensation. For the two amine-functionalized CNT types this results in covalent attachment of the SWCNTs in the polymer, that is, the nanotubes become a fully integrated component of the polymer, in contrast to standard composites where they are simply dispersed in the host polymer. Obviously, this removes the risk of nanotubes sliding through the polymer and the approach should thus be highly beneficial for realizing CNT polymer composites with enhanced mechanical properties.
All composites prepared in the study exhibited a nematic phase and a glass transition temperature that is similar to that of the pure host polymer (about 55°C). The melting temperature was, however, raised by about 10°C upon nanotube addition. Although optical microscopy images reveal some large-scale aggregates the authors attribute these to impurities, maintaining that the nanotubes are well integrated into the polymer matrix. Fibers were extruded from composites as well as the pure polymer in the nematic phase. Electron microscopy and X-ray characterization of the fibers revealed a generally good, well-aligned fibrillar internal structure, for CNT-containing as well as pristine polymer. The fibers were subjected to mechanical testing, prior to and after thermal annealing. In particular, the samples with covalently integrated nanotubes showed substantial improvements of 80–100% in tensile strength (250 MPa or 270 MPa for the unannealed/annealed pristine polymer and 346 Mpa and 629 MPa for the unannealed/annealed composite with phenylenediamine-functionalized tubes) and up to 60% reduction of the elongation at break. The simple carboxylate-functionalized nanotubes also yielded an improvement compared to the pristine polymer, but of lower magnitude, 40% and 30%, respectively. The elastic modulus was improved from 7.1 GPa (no annealing) or 12.8 Gpa (annealed) for the pristine polymer to around 12 GPa (no annealing) or around 14 GPa (annealed) upon nanotube addition, in this case with very similar results for the carboxylate-functionalized tubes and the tubes that were covalently grafted to the polymer.
Hu et al. functionalized multiwall, poly{6- [4-(4-methoxyphenylazo)phenoxy]hexyl methacrylate carbon nanotubes covalently with side-chain azobenzene liquid crystalline polymer (PMMAZO) and studied the properties of the liquid crystal phase formed by the resulting substance on its own [160]. The phase sequence of the CNT–PMMAZO is similar to that of pristine PMMAZO, with a nematic as well as a smectic phase in the mesophase temperature range, although the nematic–smectic transition peak picked up in DSC is substantially smaller for the nanotube-containing material than the pristine liquid crystalline polymer. Conductivity measurements under electric field suggest that the nanotubes are aligned to some extent, but this is in fact seen also when the system is in its isotropic phase. The results are thus somewhat inconclusive. Considering that the system contains a very high degree of carbon nanotubes more clear-cut results might be expected from a polarizing microscopy investigation of an aligned sample, relying on the anisotropic absorption of aligned nanotubes, as was previously done for nanotubes aligned in lyotropic liquid crystals [66, 67].
As discussed above the covalent modification of the nanotubes can in some cases be avoided by adding appropriate mediating molecules. These should adsorb non-covalently to the CNTs and provide it with an outer surface that integrates well with the liquid crystal polymer matrix. Among the best approaches is to use a mediating molecule that itself is a liquid crystal polymer. For instance, Ji et al. designed and synthesized a nematic main-chain liquid crystal polymer that was pyrene-terminated at both ends, providing anchor groups to carbon nanotubes [93]. The new polymer seems to provide a remarkable stabilization of nanotubes dispersed in organic solvents. According to the authors up to 12 mg/mL nanotubes can be dispersed, although there are no data for the dispersion quality and procedure for preparing the dispersion at such concentration. At 1 mg/mL concentration the authors find that simple stirring can produce a dispersion where the nanotube aggregates are below optical size. For better results sonication is however required. The pyrene-functionalized liquid crystal polymer was used for preparing monodomain liquid crystal elastomer samples, which can work as actuators [15, 161, 162], with MWCNTs added at a concentration between 0.03 wt.% and 3 wt.%. No images of the resulting composites were shown, making it difficult to judge the quality of the dispersion, but the alignment of the nanotubes within the matrix was verified by terahertz spectroscopy. An anisotropic absorption was obtained for the higher carbon nanotube loadings, the absorption coefficient being almost twice as large for polarization along the director as for the perpendicular polarization, suggesting nanotube alignment along the director in the nematic elastomer. As the nanotube concentration is quite high in this work, the alignment of the nanotube actually ought to be easy to verify optically, just by studying the absorption of polarized light.
Liquid crystalline elastomers, extensively studied by the Terentjev's group, were the target host also in the work of Yang et al., where they used poly(p-phenyleneethynylene) as added mediator for dispersing single-wall carbon nanotubes without covalent modification [163]. In this work the alignment of the nanotubes was not a prime issue but scanning electron microscopy of the surface indicates a relatively uniform orientation along the liquid crystal director. The function of the CNTs was to absorb infrared light and to transfer the energy as heat to the elastomer matrix. The CNTs thus acted as tiny distributed heaters, rapidly taking the elastomer above the nematic–isotropic transition temperature upon IR irradiation and thereby initiating the actuation.
An example of the third approach, where the liquid crystal polymer is tailored for compatibility with CNTs, is provided by Sordi et al. [164]. They incorporated sulfonate groups along the backbone of a liquid crystalline polyester in order to make it more compatible with carbon nanotubes or with nanodiamond. These nanoparticles were added under sonication at 1 wt.% to the polymer dissolved in a mixture of dichloromethane and trifluoracetic acid. Significant changes in the glass transition temperature upon addition of the nanoparticles to the sulfonated polymer indicate interactions between guests and hosts and some improvements of the mechanical properties were found, in particular with added nanodiamond. However, optical microscopy reveal very strong nanotube aggregation within the matrix, hence the procedure obviously calls for further optimization. Possibly in situ polymerization with the nanotubes added may lead to better results.
Cervini et al. explored a slightly different route, working with a low-molar mass liquid crystal host but of a polymerizable type [165]. This allowed them to turn the nanotube-liquid crystal composite into a solid film, with retained uniaxial orientation of the nanotubes. The multi-wall nanotubes had first been oxidized for adding hydroxy and carboxylic groups to their surfaces, allowing a subsequent attachment of aminopropyltriethoxysilane (APTES) moieties for improved incorporation in the liquid crystalline host and attachment to the glass substrate used for sample preparation. The authors point out that for multiwall nanotubes the oxidation may actually even be beneficial for the conductive properties, since sp3 interwall connections are created in the process. The tubes were added at concentrations up to 5 wt.% into a mixture of polymerizable low-molar mass mesogens with acrylate side groups, with ethanol added as solvent, and dispersed by means of ultrasonication. After homogenization and alignment of the liquid crystal in a homeotropic geometry the sample was photopolymerized by UV light irradiation.
The APTES-functionalized nanotubes were much better dispersed than the tubes carrying only carboxylate groups. Electron microscopy and spectroscopy suggest that the tubes were well dispersed and aligned along the homeotropic liquid crystal director, although the description of the spectroscopy is not fully convincing. It is unclear how the measurements along and perpendicular to the director were made and if the data are really reliable because the sample alignment was hometropic and not with uniform in-plane director. This means that the light beam must have propagated along the film for the measurement with polarization parallel to the director but perpendicular for the other measurement. Apart from the difficulties in realizing such measurement geometries, the sample thickness for the two cases comes out dramatically different, hence it is not easy to compare the data. The description of the optical experiments is unfortunately rather bewildering and does not at all address these important details. More convincing is the study of the electrical properties which compares the in-plane and perpendicular conductivity of the composite film. The perpendicular conductivity is substantially greater than for the in-plane geometry, in particular above CNT concentrations of about 1 wt.%, which thus seems to constitute the percolation threshold. This indeed suggests that the tubes were aligned homeotropically by the liquid crystal and that the alignment was not lost in the polymerization process.
10.7 Conclusions and Outlook
Liquid crystals have proven, and are continuously showing, to be very interesting for carbon nanotube research and for finding a way toward the application of this new nanoparticle class. The nanotube organization, critical for their successful, macroscopic use, can be achieved through the induction of LC phases from the CNTs or by mixing them with thermotropic or lyotropic LCs. Both classes of LCs have proven to be very efficient for aligning CNTs transferring their order. Solid materials can be realized with liquid crystal polymers or polymerizable LCs, enlarging the attractiveness of LCs.
The dispersion of CNTs is a critical issue for the CNT community, prerequisite for any further development. One way to solve it is with the use of surfactants. This approach makes lyotropic LCs phases particularly attractive for realizing composites with high loading of well dispersed CNTs. On the other hand tailored research has been devoted towards the dispersion of CNTs in thermotropics as well as polymer-based LCs finding suitable additives and/or evaluating the impact of the molecular structure on the quality of the dispersion. The encouraging results open new attractive ways for good dispersion of CNTs with these materials and call for more research on the topic. Improvements of the properties of composites can be expected but also novel uses. Furthermore, investigations are still required concerning the CNT–LC interaction and the properties of the combination. Interestring developments can come using tubes fractionated according to their type and dimensions, beneficial not only for the formation of LC phases from CNTs but also for the CNTs in the different LC matrices.
Liquid crystals, with their different types and the enormous variety of molecular structures or constituents provide a rich playground for creating new performing materials, devices or new superstructures based on CNTs, allowing to highlight their exceptional properties. The many diverse studies on LCs and CNTs have indicated the broadness of the subject that can be considered nowadays an autonomous research topic all around. The solid results and promising findings open new avenues of research and promise new exciting science and applications.
1. J. P. F. Lagerwall and G. Scalia. Carbon nanotubes in liquid crystals (Feature article). J. Mater. Chem. 2008, 18, 2890–2898.
2. M. Rahman and W. Lee. Scientific duo of carbon nanotubes and nematic liquid crystals. J. Phys. D-Appl. Phys. 2009, 42, 063001.
3. G. Scalia. Alignment of carbon nanotubes in thermotropic and lyotropic liquid crystals. ChemPhysChem 2010, 11, 333–340.
4. P. Brochu and Q. Pei. Advances in dielectric elastomers for actuators and artificial muscles. Macromol. Rapid Commun. 2010, 31, 10–36.
5. H. K. Bisoyi and S. Kumar. Liquid-crystal nanoscience: an emerging avenue of soft self-assembly. Chem. Soc. Rev. 2011, 40, 306–319.
6. M. Supova, G. S. Martynkova, and K. Barabaszova. Effect of nanofillers dispersion in polymer matrices: A review. Science Adv. Mater 2011, 3, 1–25.
7. S. J. Zhang and S. Kumar. Carbon nanotubes as liquid crystals. Small 2008, 4, 1270–1283.
8. S. Iijima. Helical microtubules of graphitic carbon. Nature 1991, 354, 56–58.
9. R. Saito, M. S. Dresselhaus, and G. Dresselhaus. Physical Properties of Carbon Nanotubes. Imperial College Press, 1998.
10. R. H. Baughman, A. A. Zakhidov, and W. A. de Heer. Carbon nanotubes – the route toward applications. Science 2002, 297, 787–792.
11. B. Vigolo, A. Penicaud, C. Coulon, C. Sauder, R. Pailler, C. Journet, P. Bernier, and P. Poulin. Macroscopic fibers and ribbons of oriented carbon nanotubes. Science 2000, 290, 1331–1334.
12. R. Haggenmueller, H.H. Gommans, A.G. Rinzler, J.E. Fischer, and K.L. Winey. Aligned single-wall carbon nanotubes in composites by melt processing methods. Chem. Phys. Lett. 2000, 330, 219–225.
13. E. S. Choi, J. S. Brooks, D. L. Eaton, M. S. Al-Haik, M. Y. Hussaini, H. Garmestani, D. Li, and K. Dahmen. Enhancement of thermal and electrical properties of carbon nanotube polymer composites by magnetic field processing. J. Appl. Phys. 2003, 94, 6034–6039.
14. M. Zhang, S. Fang, A. A. Zakhidov, S. B. Lee, A. E. Aliev, C. D. Williams, K. R. Atkinson, and R.H. Baughman. Strong, transparent, multifunctional, carbon nanotube sheets. Science 2005, 309, 1215–1219.
15. S. Ahir and E. M. Terentjev. Photomechanical actuation in polymer–nanotube composites. Nature Mater. 2005, 4, 491–495.
16. J. Guo, S. Hassan, A. Javey, G. Bosman, and M. Lundstrom. Assessment of high-frequency performance potential of carbon nanotube transistors. IEEE Transactions on Nanotechnology 2005, 4, 715–721.
17. H. Ko and V. Tsukruk. Liquid-crystalline processing of highly oriented carbon nanotube arrays for thin-film transistors. Nano Lett. 2006, 6, 1443–1448.
18. S. J. Kang, C. Kocabas, T. Ozel, M. Shim, N. Pimparkar, M. A. Alam, S. V. Rotkin, and J. A. Rogers. High-performance electronics using dense, perfectly aligned arrays of single-walled carbon nanotubes. Nat. Nanotechnol. 2007, 2, 230–236.
19. Iakoubovskii, K. Techniques of aligning carbon nanotubes. Central Eur. J. Phys. 2009, 7, 645–653.
20. T. Druzhinina, S. Hoeppener, and U. Schubert. Strategies for post-synthesis alignment and immobilization of carbon nanotubes. Adv. Mater. 2011, 23, 953–970.
21. S. M. Huang, L. M. Dai, and A. W. H. Mau. Patterned growth and contact transfer of well-aligned carbon nanotube films. J. Phys. Chem. B 1999, 103, 4223–4227.
22. S. Fan, M. G. Chapline, N. R. Franklin, T. W. Tombler, A. M. Cassell, and H. Dai. Self-oriented regular arrays of carbon nanotubes and their field emission properties. Science 1999, 283, 512.
23. S. M. Huang, X. Y. Cai, and J. Liu. Growth of millimeter-long and horizontally aligned single-walled carbon nanotubes on flat substrates. J. Am. Chem. Soc. 2003, 125, 5636–5637.
24. Y. Murakami, S. Chiashi, Y. Miyauchi, M. Hu, M. Ogura, T. Okubo, and S. Maruyama. Growth of vertically aligned single-walled carbon nanotube films on quartz substrates and their optical anisotropy. Chem. Phys. Lett. 2004, 385, 298–303.
25. K. Bubke, H. Gnewuch, M. Hempstead, J. Hammer, and M. Green. Optical anisotropy of dispersed carbon nanotubes induced by an electric field. Appl. Phys. Lett. 1997, 71, 1906–1908.
26. K. Yamamoto, S. Akita, and Y. Nakayama, Y. Orientation and purification of carbon nanotubes using ac electrophoresis. J. Phys. D-Appl. Phys. 1998, 31, L34–L36.
27. S. Gerdes, T. Ondarcuhu, S. Cholet, and C. Joachim. Combing a carbon nanotube on a flat metal–insulator–metal nanojunction. Europhys. Lett. 1999, 48, 292–298.
28. X. Chen, T. Saito, H. Yamada, and K. Matsushige. Aligning single-wall carbon nanotubes with an alternating-current electric field. Appl. Phys. Lett. 2001, 78, 3714–3716.
29. D. A. Walters, M. J. Casavant, X. C. Qin, C. B. Huffman, P. J. Boul, L. M. Ericson, E. H. Haroz, M. J. O'Connell, K. Smith, D. T. Colbert, and R. E. Smalley. In-plane-aligned membranes of carbon nanotubes. Chem. Phys. Lett. 2001, 338, 14–20.
30. T. Kimura, H. Ago, M. Tobita, S. Ohshima, M. Kyotani, and M. Yumura. Polymer composites of carbon nanotubes aligned by a magnetic field. Adv. Mater. 2002, 14, 1380–1383.
31. J. E. Fischer, W. Zhou, J. Vavro, C. Llaguno, C. Guthy, R. Haggenmueller, M. J. Casavant, D. E. Walters, and R. E. Smalley. Magnetically aligned single wall carbon nanotube films: Preferred orientation and anisotropic transport properties. J. Appl. Phys. 2003, 93, 2157–2163.
32. R. Krupke, F. Hennrich, H. von Lohneysen, and M. M. Kappes. Separation of metallic from semiconducting single-walled carbon nanotubes. Science 2003, 301, 344–347.
33. H. Wang, G. T. Christopherson, Z. Y. Xu, L. Porcar, D. L. Ho, D. Fry, and E. K. Hobbie. Shear-sans study of single-walled carbon nanotube suspensions. Chem. Phys. Lett. 2005, 416, 182–186.
34. C. Martin, J. Sandler, A. Windle, M. Schwarz, W. Bauhofer, K. Schulte, and M. Shaffer. Electric field-induced aligned multi-wall carbon nanotube networks in epoxy composites. Polymer 2005, 46, 877–886.
35. Q. W Li, Y. T. Zhu, I. A. Kinloch, and A. H. Windle. Self-organization of carbon nanotubes in evaporating droplets. J. Phys. Chem. B 2006, 110, 13926–13930.
36. J. M. Russell, S. J. Oh, I. LaRue, O. Zhou, and E. T. Samulski. Alignment of nematic liquid crystals using carbon nanotube films. Thin Solid Films 2006, 509, 53–57.
37. I. S. Baik, S. Y. Jeon, S. H. Lee, K. A. Park, S. H. Jeong, K. H. An, and Y. H. Lee. Electrical-field effect on carbon nanotubes in a twisted nematic liquid crystal cell. Appl. Phys. Lett. 2005, 87, 263110.
38. H. Y. Chen, W. Lee, and N. A. Clark. Faster electro-optical response characteristics of a carbon-nanotube-nematic suspension. Appl. Phys. Lett. 2007, 90, 033510.
39. H. Chen and W. Lee. Suppression of field screening in nematic liquid crystals by carbon nanotubes. Appl. Phys. Lett. 2006, 88, 222105.
40. C. Huang, C. Hu, H. Pan, and K. Lo. Electrooptical responses of carbon nanotube-doped liquid crystal devices. Jpn. J. Appl. Phys. 2005, 44, 8077–8081.
41. C. Huang and H. Pan. Comment on “electric-field effect on carbon nanotubes in a twisted nematic liquid crystal cell” [appl. Phys. Lett. 87, 263110 (2005)]. Appl. Phys. Lett. 2006, 89, 056101.
42. C. Huang, H. Pan, and C. Hsieh. Electrooptical properties of carbon–nanotube-doped twisted nematic liquid crystal cell. Jpn. J. Appl. Phys. 2006, 45, 6392–6394.
43. S. Y. Jeon, S. H. Shin, S. J. Jeong, S. H. Lee, S. H. Jeong, Y. H. Lee, H. C. Choi, and K. J. Kim. Effects of carbon nanotubes on electro-optical characteristics of liquid crystal cell driven by in-plane field. Appl. Phys. Lett. 2007, 90, 121901.
44. S. Y. Jeon, S. H. Shin, J. H. Lee, S. H. Lee, and Y. H. Lee. Effects of carbon nanotubes on nematic backflow in a twisted nematic liquid-crystal cell. Jpn. J. Appl. Phys. 2007, 46, 7801–7802.
45. W. Lee, C. Wang, and Y. Shih. Effects of carbon nanosolids on the electro-optical properties of a twisted nematic liquid-crystal host. Appl. Phys. Lett. 2004, 85, 513–515.
46. G. Scalia, J. P. F. Lagerwall, S. Schymura, M. Haluska, F. Giesselmann, and S. Roth. Carbon nanotubes in liquid crystals as versatile functional materials. Physica Status Solidi B 2007, 244, 4212–4217.
47. P. van der Schoot, V. Popa-Nita, and S. Kralj. Alignment of carbon nanotubes in nematic liquid crystals. J. Phys. Chem. B 2008, 112, 4512–4518.
48. W. Gwizdala, K. Gorny, and Z. Gburski. Molecular dynamics and dielectric loss in 4-cyano-4-n-pentylbiphenyl (5CB) mesogene film surrounding carbon nanotube - Computer simulation. J. Mol. Structure 2008, 887, 148–151.
49. F. R. Hung. Quadrupolar particles in a nematic liquid crystal: Effects of particle size and shape. Phys. Rev. E 2009, 79, 021705.
50. A. Dawid and W. Gwizdala. Dynamical and structural properties of 4-cyano-4-n-pentylbiphenyl (5CB) molecules adsorbed on carbon nanotubes of different chiralities: Computer simulation. J. Non-crystalline Solids 2009, 355, 1302–1306.
51. M. J. Green, A. N. G. Parra-Vasquez, N. Behabtu, and M. Pasquali. Modeling the phase behavior of polydisperse rigid rods with attractive interactions with applications to single-walled carbon nanotubes in superacids. J. Chem. Phys. 2009, 131, 084901.
52. V. Popa-Nita and S. Kralj. Liquid crystal-carbon nanotubes mixtures. J. Chem. Phys. 2010, 132, 024902.
53. A. Matsuyama. Biaxial nematic phase in mixtures of a liquid crystal and a rodlike polymer. Mol. Cryst. Liq. Cryst. 2011, 540, 42–49.
54. A. Matsuyama. Biaxial nematic phases in rod/liquid crystal mixtures. Liq. Cryst. 2011, 38, 729–736.
55. A. M. Somoza, C. Sagui, and C. Roland. Liquid crystal phases of capped carbon nanotubes. Phys. Rev. B 2001, 63, 81403. (R).
56. S. Giordani, S. D. Bergin, V. Nicolosi, S. Lebedkin, M. M. Kappes, W. J. Blau, and J. N. Coleman. Debundling of single-walled nanotubes by dilution: Observation of large populations of individual nanotubes in amide solvent dispersions. J. Phys. Chem. B. 2006, 110, 15708–15718.
57. S. D. Bergin, Z. Y. Sun, D. Rickard, P. V. Streich, J. P. Hamilton, and J. N. Coleman. Multicomponent solubility parameters for single-walled carbon nanotube-solvent mixtures. ACS Nano 2009, 3, 2340–2350.
58. H. Wang. Dispersing carbon nanotubes using surfactants. Curr. Opin. Colloid Interface Sci. 2009, 14, 364–371.
59. M. Zheng, A. Jagota, E. D. Semke, B. A. Diner, R. S. Mclean, S. R. Lustig, R. E. Richardson, and N. G. Tassi. DNA-assisted dispersion and separation of carbon nanotubes. Nature Mater. 2003, 2, 338–342.
60. S. Park, H. S. Yang, D. Kim, K. Jo, and S. Jon. Rational design of amphiphilic polymers to make carbon nanotubes water-dispersible, anti-biofouling, and functionalizable. Chem. Commun. 2008, 2876–2878.
61. S. Schymura, S. Dölle, J. Yamamoto, and J. Lagerwall. Filament formation in carbon nanotube-doped lyotropic liquid crystals. Soft Matter 2011, 7, 2663–2667.
62. F. Tardani and L. M. Camillo. Elasticity of dispersions based on carbon nanotubes dissolved in a lyotropic nematic solvent. J. Phys. Chem. C 2011, 115, 9424–9431.
63. X. Xin, H. Li, E. Kalwarczyk, A. Kelm, M. Fialkowski, E. Gorecka, D. Pociecha, and R. Holyst. Single-walled carbon nanotube/lyotropic liquid crystal hybrid materials fabricated by a phase separation method in the presence of polyelectrolyte. Langmuir 2010, 26, 8821–8828.
64. Y. S. Kwon, B. M. Jung, H. Lee, and J. Y. Chang. Preparation of polymeric SWNT-liquid crystal composites using a polymerizable surfactant. Macromolecules 2010, 43, 5376–5381.
65. X. Xin, H. Li, S. A. Wieczorek, T. Szymborski, E. Kalwarczyk, N. Ziebacz, E. Gorecka, D. Pociecha, and R. Holyst. Incorporation of carbon nanotubes into a lyotropic liquid crystal by phase separation in the presence of a hydrophilic polymer. Langmuir 2010, 26, 3562–3568.
66. S. Schymura, E. Enz, S. Roth, G. Scalia, and J. P. F. Lagerwall. Macroscopic-scale carbon nanotube alignment via self-assembly in lyotropic liquid crystals. Synthetic Metals 2009, 159, 2177–2179.
67. G. Scalia, C. von Bühler, C. Hägele, S. Roth, F. Giesselmann, and J. P. F. Lagerwall. Spontaneous macroscopic carbon nanotube alignment via colloidal suspension in hexagonal columnar lyotropic liquid crystals. Soft Matter 2008, 4, 570–576.
68. C. Zamora-Ledezma, C. Blanc, M. Maugey, C. Zakri, P. Poulin, and E. Anglaret. Anisotropic thin films of single-wall carbon nanotubes from aligned lyotropic nematic suspensions. Nano Lett. 2008, 8, 4103–4107.
69. J. P. F. Lagerwall, G. Scalia, M. Haluska, U. Dettlaff-Weglikowska, S. Roth, and F. Giesselmann. Nanotube alignment using lyotropic liquid crystals. Adv. Mater. 2007, 19, 359–364.
70. V. Weiss, R. Thiruvengadathan, and O. Regev. Preparation and characterization of a carbon nanotube-lyotropic liquid crystal composite. Langmuir 2006, 22, 854–856.
71. J. P. F. Lagerwall, G. Scalia, M. Haluska, U. Dettlaff-Weglikowska, S. Roth, and F. Giesselmann. Simultaneous alignment and dispersion of carbon nanotubes with lyotropic liquid crystals. Phys. Status Solidi B 2006, 243, 3046–3049.
72. O. Mondain-monval and P. Poulin. Mixed colloid-surfactant systems. J. Phys. Condensed Matter 2004, 16, S1873–S1885.
73. M. Zapotocky, L. Ramos, P. Poulin, T. C. Lubensky, and D. A. Weitz. Particle-stabilized defect gel in cholesteric liquid crystals. Science 1999, 283, 209–212.
74. C. Zakri and P. Poulin. Phase behavior of nanotube suspensions: From attraction induced percolation to liquid crystalline phases. J. Mater. Chem. 2006, 16, 4095–4098.
75. E. Nativ-Roth, R. Yerushalmi-Rozen, and O. Regev. Phase behavior and shear alignment in SWNT-surfactant dispersions. Small 2008, 4, 1459–1467.
76. E. Nativ-Roth, O. Regev, and R. Yerushalmi-Rozen. Shear-induced ordering of micellar arrays in the presence of single-walled carbon nanotubes. Chem. Commun. 2008, 2037–2039.
77. E. Nativ-Roth, R. J. Nap, I. Szleifer, and R. Yerushalmi-Rozen. Order–disorder transition induced by surfactant micelles in single-walled carbon nanotubes dispersions. Soft Matter 2010, 6, 5289–5292.
78. N. Nakashima, S. Okuzono, H. Murakami, T. Nakai, and K. Yoshikawa. DNA dissolves single-walled carbon nanotubes in water. Chem. Lett. 2003, 32, 456–457.
79. M. Zheng, A. Jagota, M. S. Strano, A. P. Santos, P. Barone, S.G. Chou, B. A. Diner, M. S. Dresselhaus, R. S. McLean, G. B. Onoa, G. G. Samsonidze, E. D. Semke, M. Usrey, and D. J. Walls. Structure-based carbon nanotube sorting by sequence-dependent DNA assembly. Science 2003, 302, 1545–1548.
80. S. Badaire, C. Zakri, M. Maugey, A. Derre, J. N. Barisci, G. Wallace, and P. Poulin. Liquid crystals of DNA-stabilized carbon nanotubes. Adv. Mater. 2005, 17, 1673.
81. B. Gigliotti, B. Sakizzie, D. Bethune, R. Shelby, and J. Cha. Sequence-independent helical wrapping of singles-walled carbon nanotubes by long genomic DNA. Nano Lett. 2006, 6, 159–164.
82. H. Cathcart, S. Quinn, V. Nicolosi, J. M. Kelly, W. J. Blau, and J. N. Coleman. Spontaneous debundling of single-walled carbon nanotubes in DNA-based dispersions. J. Phys. Chem. C 2007, 111, 66–74.
83. A. N. Enyashin, S. Gemming, and G. Seifert. DNA-wrapped carbon nanotubes. Nanotechnology 2007, 18, 245702.
84. R. R. Johnson, A. T. C. Johnson, and M. L. Klein. Probing the structure of DNA–Carbon nanotube hybrids with molecular dynamics. Nano Lett. 2007, 8, 69–75.
85. X. Tu and M. Zheng. A DNA-based approach to the carbon nanotube sorting problem. Nano Res. 2008, 1, 185–194.
86. G. Y. Ao, D. Nepal, M. Aono, and V. A. Davis. Cholesteric and nematic liquid crystalline phase behavior of double-stranded DNA stabilized single-walled carbon nanotube dispersions. ACS Nano 2011, 5, 1450–1458.
87. W. Wenseleers, I. I. Vlasov, E. Goovaerts, E. D. Obraztsova, A. S. Lobach, and A. Bouwen. Efficient isolation and solubilization of pristine single-walled nanotubes in bile salt micelles. Adv. Funct. Mater. 2004, 14, 1105–1112.
88. N. Puech, E. Grelet, P. Poulin, C. Blanc, and P. van der Schoot. Nematic droplets in aqueous dispersions of carbon nanotubes. Phys. Rev. E 2010, 82, 020702.
89. J. P. F. Lagerwall, R. Dabrowski, and G. Scalia. Antiferroelectric liquid crystals with induced intermediate polar phases and the effects of doping with carbon nanotubes. J. Non-crystalline Solids 2007, 353, 4411–4417.
90. S. Schymura, M. Kühnast, V. Lutz, S. Jagiella, U. Dettlaff-Weglikowska, S. Roth, F. Giesselmann, C. Tschierske, G. Scalia, and J. Lagerwall. Towards efficient dispersion of carbon nanotubes in thermotropic liquid crystals. Adv. Funct. Mater. 2010, 20, 3350–3357.
91. G. Scalia, J. P. F. Lagerwall, M. Haluska, U. Dettlaff-Weglikowska, F. Giesselmann, and S. Roth. Effect of phenyl rings in liquid crystal molecules on SWCNTs studied by Raman spectroscopy. Physica Status Solidi B 2006, 243, 3238–3241.
92. S. Meuer, L. Braun, and R. Zentel. Solubilisation of multi walled carbon nanotubes by alpha-pyrene functionalised PMMA and their liquid crystalline self-organisation. Chem. Commun. 2008, 3166–3168.
93. Y. Ji, Y. Y. Huang, R. Rungsawang, and E. M. Terentjev. Dispersion and alignment of carbon nanotubes in liquid crystalline polymers and elastomers. Adv. Mater. 2010, 22, 3436–3440.
94. M. Kühnast, C. Tschierske, and J. Lagerwall. Tailor-designed polyphilic promotors for stabilizing dispersions of carbon nanotubes in liquid crystals. Chem. Commun. 2010, 6989–6991.
95. M. Kimura, N. Miki, N. Adachi, Y. Tatewaki, K. Ohta, and H. Shirai. Organization of single-walled carbon nanotubes wrapped with liquid-crystalline-conjugated oligomers. J. Mater. Chem. 2009, 19, 1086–1092.
96. S. Manne, J. Cleveland, H. Gaub, G. Stucky, and P. Hansma. Direct visualization of surfactant Hemimicelles by force microscopy of the electrical double-layer. Langmuir 1994, 10, 4409–4413.
97. H. W. Lee, W. You, S. Barman, S. Hellstrom, M. C. LeMieux, J. H. Oh, S. Liu, T. Fujiwara, W. M. Wang, B. Chen, Y. W. Jin, J. M. Kim, and Z. Bao. Lyotropic liquid-crystalline solutions of high-concentration dispersions of single-walled carbon nanotubes with conjugated polymers. Small 2009, 5, 1019–1024.
98. O. Trushkevych, N. Collings, T. Hasan, V. Scardaci, A. C. Ferrari, T. D. Wilkinson, W. A. Crossland, W. I. Milne, J. Geng, B. F. G. Johnson, and S. Macaulay. Characterization of carbon nanotube-thermotropic nematic liquid crystal composites. J. Phys. D-Appl. Phys. 2008, 41, 125106.
99. L. Onsager. The effects of shape on the interaction of colloidal particles. Ann. N. Y. Acad. Sci. 1949, 51, 627–659.
100. A. Donald, A. Windle, and S. Hanna. Liquid Crystalline Polymers. Cambridge University Press, 2006.
101. W. H. Song, I. A. Kinloch, and A. H. Windle. Nematic liquid crystallinity of multiwall carbon nanotubes. Science 2003, 302, 1363–1363.
102. W. Song and A. Windle. Isotropic-nematic phase transition of dispersions of multiwall carbon nanotubes. Macromolecules 2005, 38, 6181–6188.
103. S. J. Zhang, I. A. Kinloch, and A. H. Windle. Mesogenicity drives fractionation in lyotropic aqueous suspensions of multiwall carbon nanotubes. Nano Lett. 2006, 6, 568–572.
104. S. E. Moulton, M. Maugey, P. Poulin, and G. G. Wallace. Liquid crystal behavior of single-walled carbon nanotubes dispersed in biological hyaluronic acid solutions. J. Am. Chem. Soc. 2007, 129, 9452–9457.
105. V. Davis, L. Ericson, A. Parra-vasquez, H. Fan, Y. Wang, V. Prieto, J. Longoria, S. Ramesh, R. Saini, C. Kittrell, W. Billups, W. Adams, R. Hauge, R. Smalley, and M. Pasquali. Phase behavior and rheology of SWNTs in superacids. Macromolecules 2004, 37, 154–160.
106. L. M. Ericson, H. Fan, H. Peng, V. A. Davis, W. Zhou, J. Sulpizio, Y. Wang, R. Booker, J. Vavro, C. Guthy, A. N. Parra-Vasquez, M. J. Kim, S. Ramesh, R. K. Saini, C. Kittrell, G. Lavin, H. Schmidt, W. W. Adams, W. E. Billups, M. Pasquali, W. F. Hwang, R. H. Hauge, J. E. Fischer, and R. E. Smalley. Macroscopic, neat, single-walled carbon nanotube fibers. Science 2004, 305, 1447–1450.
107. P. Rai, R. Pinnick, A. Parra-vasquez, V. Davis, H. Schmidt, R. Hauge, R. Smalley, and M. Pasquali. Isotropic-nematic phase transition of single-walled carbon nanotubes in strong acids. J. Am. Chem. Soc. 2006, 128, 591–595.
108. N. Puech, C. Blanc, E. Grelet, C. Zamora-Ledezma, M. Maugey, C. Zakri, E. Anglaret, and P. Poulin. Highly ordered carbon nanotube nematic liquid crystals. J. Phys. Chem. C 2011, 115, 3272–3278.
109. V. A. Davis, A. N. G. Parra-Vasquez, M. J. Green, P. K. Rai, N. Behabtu, V. Prieto, R. D. Booker, J. Schmidt, E. Kesselman, W. Zhou, H. Fan, W. W. Adams, R. H. Hauge, J. E. Fischer, Y. Cohen, Y. Talmon, R. E. Smalley, and M. Pasquali. True solutions of single-walled carbon nanotubes for assembly into macroscopic materials. Nat. Nanotechnol. 2009, 4, 830–834.
110. A. N. G Parra-Vasquez, N. Behabtu, M. J. Green, C. L. Pint, C. C. Young, J. Schmidt, E. Kesselman, A. Goyal, P. M. Ajayan, Y. Cohen, Y. Talmon, R. H. Hauge, and M. Pasquali. Spontaneous dissolution of ultralong single- and multiwalled carbon nanotubes. ACS Nano 2010, 4, 3969–3978.
111. W. H. Song and A. H. Windle. Size-dependence and elasticity of liquid-crystalline multiwalled carbon nanotubes. Adv. Mater. 2008, 20, 3149–3154.
112. A. Speranza and P. Sollich. Isotropic–nematic phase equilibria in the Onsager theory of hard rods with length polydispersity. Phys. Rev. E. 2003, 67, 061702.
113. S. Zhang, Q. Li, I. A. Kinloch, and A. H. Windle. Ordering in a droplet of an aqueous suspension of single-wall carbon nanotubes on a solid substrate. Langmuir 2010, 26, 2107–2112.
114. R. Wang, J. Sun, and L. Gao. Liquid-crystal phase reinforced carbon nanotube fibers. J. Phys. Chem. C 2010, 114, 4923–4928.
115. S. J. Zhang, K. K. K. Koziol, I. A. Kinloch, and A. H. Windle. Macroscopic fibers of well-aligned carbon nanotubes by wet spinning. Small 2008, 4, 1217–1222.
116. M. Islam, A. Alsayed, Z. Dogic, J. Zhang, T. Lubensky, and A. Yodh. Nematic nanotube gels. Phys. Rev. Lett. 2004, 92, 088303.
117. F. Brochard and P.-G. de Gennes. Theory of magnetic suspensions in liquid crystals. J. Phys. (Paris) 1970, 31, 691–708.
118. S. V. Burylov and Y. L. Raikher. Orientation of a solid particle embedded in a monodomain nematic liquid crystal. Phys. Rev. E 1994, 50, 358–367.
119. I. C. Khoo, J. Ding, Y. Y. Zhang, K. Chen, and A. Diaz. Supra-nonlinear photorefractive response of single-walled carbon nanotube- and C60-doped nematic liquid crystal. Appl. Phys. Lett. 2003, 82, 3587.
120. W. Lee, H.-Y. Chen, and S.-L. Yeh. Surface-sustained permanent gratings in nematic liquid crystals doped with carbon nanotubes. Opt. Express 2002, 10, 482–487.
121. W. Lee and S.-L. Yeh. Optical amplification in nematics doped with carbon nanotubes. Appl. Phys. Lett. 2001, 79, 4488.
122. H. J. Shah, A. K. Fontecchio, D. Mattia, and Y. Gogotsi. Field controlled nematic-to-isotropic phase transition in liquid crystal–carbon nanotube composites. J. Appl. Phys. 2008, 103, 064314.
123. R. Basu and G. S. Iannacchione. Carbon nanotube dispersed liquid crystal: a nano electromechanical system. Appl. Phys. Lett. 2008, 93, 183105.
124. A. I. Goncharuk, N. I. Lebovka, L. N. Lisetski, and S. S. Minenko. Aggregation, percolation and phase transitions in nematic liquid crystal EBBA doped with carbon nanotubes. J. Phys. D-Appl. Phys. 2009, 42, 165411.
125. L. N. Lisetski, S. S. Minenko, A. V. Zhukov, P. P. Shtifanyuk, and N. I. Lebovka. Dispersions of carbon nanotubes in cholesteric liquid crystals. Mol. Cryst. Liq. Cryst. 2009, 510, 43–50.
126. R. Basu and G. S. Iannacchione. Nematic anchoring on carbon nanotubes. Appl. Phys. Lett. 2009, 95, 173113.
127. R. Basu and G. S. Iannacchione. Dielectric hysteresis, relaxation dynamics, and nonvolatile memory effect in carbon nanotube dispersed liquid crystal. J. Appl. Phys. 2009, 106, 124312.
128. V. Jayalakshmi and S. K. Prasad. Understanding the observation of large electrical conductivity in liquid crystal-carbon nanotube composites. Appl. Phys. Lett. 2009, 94, 202106.
129. P. Arora, A. Mikulko, F. Podgornov, and W. Haase. Dielectric and electro-optic properties of new ferroelectric liquid crystalline mixture doped with carbon nanotubes. Mol. Cryst. Liq. Cryst. 2009, 502, 1–8.
130. F. V. Podgornov, A. M. Suvorova, A. V. Lapanik, and W. Haase. Electrooptic and dielectric properties of ferroelectric liquid crystal/single walled carbon nanotubes dispersions confined in thin cells. Chem. Phys. Lett. 2009, 479, 206–210.
131. L. A. Dolgov, N. I. Lebovka, and O. V. Yaroshchuk. Effect of electrooptical memory in suspensions of carbon nanotubes in liquid crystals. Colloid J. 2009, 71, 603–611.
132. O. Yaroshchuk, S. Tomylko, L. Dolgov, T. Semikina, and O. Koyalchuk. Carbon nanotubes doped liquid crystals: robust composites with a function of electro-optic memory. Diamond Relat. Mater. 2010, 19, 567–572.
133. S. K. Shriyan and A. K. Fontecchio. Analysis of effects of oxidized multiwalled carbon nanotubes on electro-optic polymer/liquid crystal thin film gratings. Opt. Express 2010, 18, 24842–24852.
134. V. V. Ponevchinsky, A. I. Goncharuk, V. I. Vasil'ev, N. I. Lebovka, and M. S. Soskin. Cluster self-organization of nanotubes in a nematic phase: the percolation behavior and appearance of optical singularities. JETP Lett. 2010, 91, 241–244.
135. M. E. Abbasov, S. Ghosh, A. Quach, and G. O. Carlisle. Hybrid carbon nanotube and dye-doped liquid crystal material for holographic imaging. J. Mater. Sci. Mater. Electron. 2010, 21, 854–859.
136. S. Tomylko, L. Dolgov, T. Semikina, and O. Yaroshchuk. Liquid crystal–carbon nanotubes composites with the induced chirality: The way towards enhancement of electro-optic memory. Mol. Cryst. Liq. Cryst. 2010, 527, 130–136.
137. O. Buluy, S. Nepijko, V. Reshetnyak, E. Ouskova, V. Zadorozhnii, A. Leonhardt, M. Ritschel, G. Schoenhense, and Y. Reznikov. Magnetic sensitivity of a dispersion of aggregated ferromagnetic carbon nanotubes in liquid crystals. Soft Matter 2011, 7, 644–649.
138. L. N. Lisetski, S. S. Minenko, V. V. Ponevchinsky, M. S. Soskin, A. I. Goncharuk, and N. I. Lebovka. Microstructure and incubation processes in composite liquid crystalline material (5CB) filled with multi walled carbon nanotubes. Materialwissenschaft Und Werkstofftechnik 2011, 42, 5–14.
139. K. P. Sigdel and G. S. Iannacchione. Effect of carbon nanotubes on the isotropic to nematic and the nematic to smectic-A phase transitions in liquid crystal and carbon nanotubes composites. Eur. Phys. J. E 2011, 34, 34.
140. K. Won, R. Rajasekharan, P. Hands, Q. Dai, and T. W. Wilkinson. Adaptive lenticular lens array using a hybrid liquid crystal-carbon nanotube nanophotonic device. Opt. Eng. 2011, 50, 054002.
141. H. S. Jeong, Y. K. Ko, Y. H. Kim, D. K. Yoon, and H.-T. Jung. Self assembled plate-like structures of single-walled carbon nanotubes by non-covalent hybridization with smectic liquid crystals. Carbon 2010, 48, 774–780.
142. R. Basu, K. A. Boccuzzi, S. Ferjani, and C. Rosenblatt. Carbon nanotube-induced chirality in an achiral liquid crystal. Appl. Phys. Lett. 2010, 97, 121908.
143. R. Basu, R. G. Petschek, and C. Rosenblatt. Nematic electroclinic effect in a carbon-nanotube-doped achiral liquid crystal. Phys. Rev. E 2011, 83, 041707.
144. R. Basu, C.-L. Chen, and C. Rosenblatt. Carbon nanotube-induced macroscopic helical twist in an achiral nematic liquid crystal. J. Appl. Phys. 2011, 109, 083518.
145. S. Kumar and H. Bisoyi. Aligned carbon nanotubes in the supramolecular order of discotic liquid crystals. Angew. Chem. Int. Ed. 2007, 46, 1501–1503.
146. M. Lynch and D. Patrick. Organizing carbon nanotubes with liquid crystals. Nano Lett. 2002, 2, 1197–1201.
147. I. Dierking, G. Scalia, and P. Morales. Liquid crystal–carbon nanotube dispersions. J. Appl. Phys. 2005, 97, 044309.
148. I. Dierking, G. Scalia, P. Morales, and D. Leclere. Aligning and reorienting carbon nanotubes with nematic liquid crystals. Adv. Mater. 2004, 16, 865–869.
149. G. Duesberg, I. Loa, M. Burghard, K. Syassen, and S. Roth. Polarized Raman spectroscopy on isolated single-wall carbon nanotubes. Phys. Rev. Lett. 2000, 85, 5436–5439.
150. G. Scalia, M. Haluska, U. Dettlaff-Weglikowska, F. Giesselmann, and S. Roth. Polarized Raman spectroscopy study of SWCNT orientational order in an aligning liquid crystalline matrix. AIP Conf. Proc. 2005, 786, 114–117.
151. S. Kumar, T. Dang, F. Arnold, A. Bhattacharyya, B. Min, X. Zhang, R. Vaia, C. Park, W. Adams, R. Hauge, R. Smalley, S. Ramesh, and P. Willis. Synthesis, structure, and properties of pbo/swnt composites. Macromolecules 2002, 35, 9039–9043.
152. J. Li, X. Chen, X. Li, H. Cao, H. Yu, and Y. Huang. Synthesis, structure and properties of carbon nanotube/poly(p-phenylene benzobisoxazole) composite fibres. Polym. Int. 2006, 55, 456–465.
153. K. Okano, I. Noguchi, and T. Yamashita. Anisotropic carbon nanotube films fabricated from a lyotropic liquid-crystalline polymer. Macromolecules 2010, 43, 5496–5499.
154. W. Q. Jiang, B. Yu, W. M. Liu, and J. C. Hao. Carbon nanotubes incorporated within lyotropic hexagonal liquid crystal formed in room-temperature ionic liquids. Langmuir 2007, 23, 8549–8553.
155. M. S. Mauter, M. Elimelech, and C. O. Osuji. Nanocomposites of vertically aligned single-walled carbon nanotubes by magnetic alignment and polymerization of a lyotropic precursor. ACS Nano 2010, 4, 6651–6658.
156. H. J. Choi, J. Y. Kim, and S. H. Kim. Multi-wall carbon nanotube reinforced thermotropic liquid crystal copolyester nanocomposites. Mol. Cryst. Liq. Cryst. 2009, 510, 300–311.
157. X. Wang, J. Wang, W. Zhao, L. Zhang, X. Zhong, R. Li, and J. Ma. Synthesis and characterization of thermotropic liquid crystalline polyester/multi-walled carbon nanotube nanocomposites. Appl. Surf. Sci. 2010, 256, 1739–1743.
158. E. Lafuente, M. Pinol, M. T. Martinez, E. Munoz, L. Oriol, and J. L. Serrano. Preparation and characterization of nematic polyazomethine/single-walled carbon nanotube composites prepared by in situ polymerization. J. Polym. Sci. Part A-Polym. Chem. 2009, 47, 2361–2372.
159. N. G. Sahoo, H. K. F. Cheng, L. Li, S. H. Chan, Z. Judeh, and J. Zhao. Specific functionalization of carbon nanotubes for advanced polymer nanocomposites. Adv. Funct. Mater. 2009, 19, 3962–3971.
160. T. Hu, H. Xie, L. Chen, G. Zhong, and H. Zhang. Preparation and orientation behavior of multi-walled carbon nanotubes grafted with a side-chain azobenzene liquid crystalline polymer. Polym. Int. 2011, 60, 93–101.
161. C. Ohm, M. Brehmer, and R. Zentel. Liquid crystalline elastomers as actuators and sensors. Adv. Mater. 2010, 22, 3366–3387.
162. S. Courty, J. Mine, A. Tajbakhsh, and E. M. Terentjev. Nematic elastomers with aligned carbon nanotubes: New electromechanical actuators. Europhys. Lett. 2008, 64, 654–660.
163. L. Q. Yang, K. Setyowati, A. Li, S. Q. Gong, and J. Chen. Reversible infrared actuation of carbon nanotube-liquid crystalline elastomer nanocomposites. Adv. Mater. 2008, 20, 2271.
164. D. Sordi, C. De Ruijter, S. Orlanducci, S. J. Picken, E. J. R. Sudhoelter, M. L. Terranova, L. C. P. M. De Smet, and T. J. Dingemans. Sulfonated liquid crystalline polyesters as resin matrix for single wall carbon nanotube and nanodiamond composites. J. Polym. Sci. Part A-Polym. Chem. 2011, 49, 1079–1087.
165. R. Cervini, G. P. Simon, M. Ginic-Markovic, J. G. Matisons, C. Huynh, and S. Hawkins. Aligned silane-treated MWCNT/liquid crystal polymer films. Nanotechnology 2008, 19, 175602.